From basalt to biosphere: Early non-vent community succession on the erupting Vailulu’u deep seamount
- 1Department of Biological Sciences, Lehigh University, Bethlehem, PA, United States
- 2Cooperative Institute for Marine Ecosystem and Resources Studies, Oregon State University, Newport, OR, United States
- 3Department of Earth Science, University of California, Santa Barbara, CA, United States
- 4Department of Earth Sciences, University of Hawai’i at Mānoa, Honolulu, HI, United States
- 5Department of Earth and Environmental Sciences, Lehigh University, Bethlehem, PA, United States
Volcanic eruptions provide rare opportunities to witness the biological recolonization of areas covered by new lava flows by effectively resetting the ecological succession clock to zero. The role of submarine volcanic eruptions as disturbance events and the resulting patterns of ecological succession have mainly been studied in hydrothermal vent ecosystems. However, the effects of submarine volcanic eruptions as disturbance forces have rarely been studied in non-vent ecosystems, particularly on seamounts. Here, we document the early stages of ecological succession of non-vent benthic communities inhabiting the summit caldera of the active Vailulu’u submarine volcano in American Samoa. Sitting above the Samoan volcanic hotspot, Vailulu’u is the youngest volcano of the Samoan chain. Repeated mapping of Vailulu’u in 1999, 2005, 2006, 2012, and 2017 revealed the progressive growth of a new cone named Nafanua. In 18 years, the cone grew >300 meters in height from a starting depth of ~1000 meters below sea level (mbsl). The differential analyses of this time-series bathymetry dataset enabled the assignment of maximum age ranges to different portions of the new cone. High-definition ROV imagery collected in 2017 revealed patterns of community structuring consistent with ecological succession: newly erupted seafloor contained a subset of the benthic species found on older seafloor. Furthermore, individual animal sizes in the younger seafloor zones were smaller than in the older zones. This unusual interdisciplinary combination of geological and biological observations provides constraints on which deep-sea animals recolonize new seafloor after a major disturbance event and how quickly. This knowledge could be applied to identify signs and states of recovery from anthropogenic disturbances by a deep seamount ecosystem.
Introduction
Understanding how the patterns of community composition change over time is a central goal in ecology (Clements, 1916; Odum, 1969). Ecological succession is the process of re-colonization and sequential replacement or change in species abundance in a community following the creation of new habitat or a disturbance event (Grime, 1979; Huston and Smith, 1987). Theoretical and empirical succession studies have focused on terrestrial ecosystems, but subtidal marine ecosystems have received far less attention, particularly in the deep sea.
Benthic communities in the deep sea are intimately tied to the geological processes that shape their habitats. New seafloor habitats are formed at the boundaries of tectonic plates or intraplate hot spots where molten lava erupts and paves over existing biological communities, thus resetting the ecological succession sequence. Most studies of primary succession (i.e., colonization of a new seafloor habitat) in the deep sea have focused on characterizing the successional dynamics in mid-ocean ridge hydrothermal vent communities after volcanic eruptions (Tunnicliffe et al., 1997; Shank et al., 1998; Marcus et al., 2009; Mullineaux et al., 2010; Gollner et al., 2015; Dykman et al., 2021). The steep nutritional and stress gradients of deep-sea hydrothermal vent environments dictate post-eruptive succession dynamics. Large, fast-growing animals with high dispersal potential dominate early successional stages. Small animals with low dispersal potential are more abundant at later stages (Shank et al., 1998; Dykman et al., 2021). However, because of the unique environmental characteristics of hydrothermal vents, it is unlikely that these primary successional dynamics can be generalized to non-vent deep-sea ecosystems.
Except for species adapted to energy-rich environments like hydrothermal vents, deep-sea animals tend to live long lives and have slow growth rates. For example, some deep-sea corals and sponges have growth rates of micrometers to millimeters per year and can live for hundreds to thousands of years (Roark et al., 2006; Roark et al., 2009; Jochum et al., 2012; Prouty et al., 2016). Due to the slow pace of biological processes in the deep sea, it is assumed that studying community change over time requires observations spanning temporal scales of years to centuries. Most of our understanding of successional dynamics in deep seamount benthic ecosystems comes from comparisons between neighboring habitats with varying degrees of anthropogenic disturbance, such as bottom trawling (Waller et al., 2007; Althaus et al., 2009; Williams et al., 2010; Baco et al., 2019; Clark et al., 2019). These comparisons indicate that disturbed deep seamount ecosystems can show signs of recovery and secondary succession (i.e., recolonization of a previously occupied habitat) over 30- to 40-year time scales (Baco et al., 2019).
Few studies have examined primary succession dynamics on new deep seafloor away from hydrothermal vents. Putts and colleagues (2019) found that deep-sea coral communities, 400 to 450 meters below sea level (mbsl) off the volcanic island of Hawai’i, show evidence of successional dynamics. Coral species diversity, abundance, and size increase as a function of the age of the seafloor they inhabit. Their study suggests that the time scales of succession in these communities span decades to millennia (the ages of the studied lava flows ranged from 61 to ~15,000 years). Milligan and Tunnicliffe (1994) found that young seafloor, 2- 6 years old, on the Cleft segment of the Juan de Fuca Ridge spreading center was scarcely colonized by sessile non-vent species. Species diversity and abundance were higher on older seafloor (> 6 years old). However, primary succession dynamics of deep seamount communities remain unknown on year-to-decade time scales, limiting our understanding of the incipient stages of colonization and development of non-vent benthic communities.
Vailulu’u, an active seamount in the territorial waters of American Samoa, is an ideal model system for understanding successional dynamics that are otherwise difficult to study in the deep sea. Vailulu’u was first mapped in March 1999 using R/V Melville’s SeaBeam2000 multibeam sonar system. This mapping survey showed that Vailulu’u is an active seamount on the Samoan volcanic hotspot (Hart et al., 2000). Vailulu’u seamount hosts a 2 km-wide summit caldera crater 400 m deeper than the caldera walls (Figure 1). Vailulu’u’s can be classified as a deep seamount as its summit is deeper than 400 m (Genin, 2004). The shallowest depth on the caldera rim is 590 m, and the deepest part of the caldera floor is 1050 m. High turbidity and chemical anomalies demonstrated hydrothermal activity inside the caldera (Hart et al., 2000; Hart et al., 2003; Staudigel et al., 2004). Ocean bottom hydrophones deployed in 2000-2001 confirmed that Vailulu’u is seismically active (Konter et al., 2004).
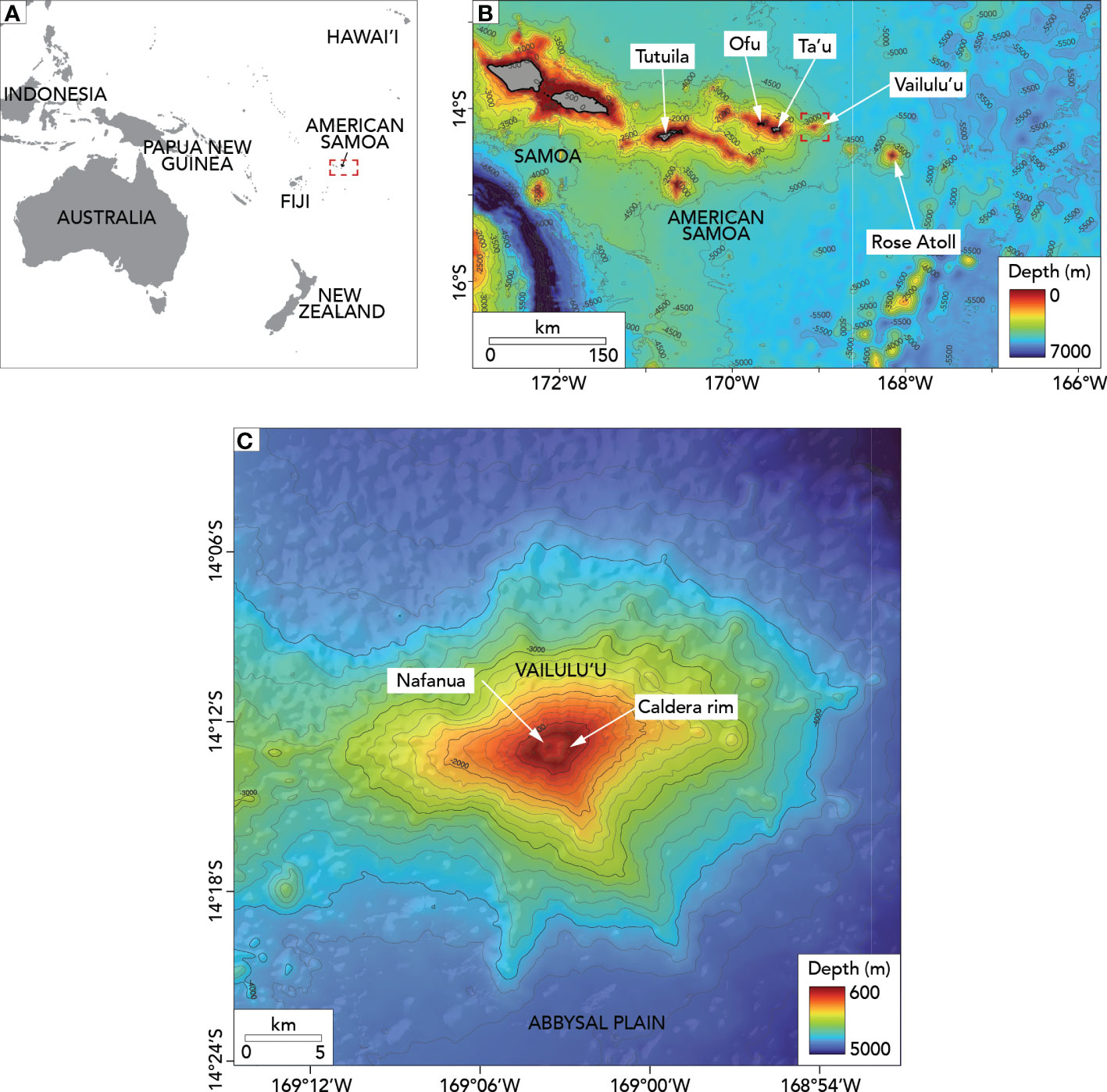
Figure 1 Maps of the study area. (A) Location of American Samoa in the Central Pacific. The red dotted box indicates the location of the Samoan region. (B) Vailulu’u seamount in the bathymetric context of the Samoan region (including the Independent State of Samoa and American Samoa). The red dotted box indicates the location of Vailulu’u seamount. (C) Bathymetry and main features of Vailulu’u. The gray lines in (B, C) show 500m and 200m depth contours, respectively.
A new 250-300-m high volcanic cone grew on the western floor of the caldera during the 6 years between 1999 and 2005 when a R/V Kilo Moana expedition re-mapped Vailulu’u using an EM120 sonar system (Staudigel et al., 2006). The new cone was named Nafanua and had a summit depth of 708 m, which is shallower than the deepest breaches in the caldera rim. Direct observations in March and July 2005 with the Pisces V submersible (R/V Ka’imika-O-Kanaloa) showed that Nafanua was formed of extensive pillow lavas that are olivine-phyric alkali basalts (Staudigel et al., 2006). The Pisces V dives also identified three hydrothermal vent fields within the caldera. Non-chemosynthetic animals were observed at and away from the hydrothermal vent fields (Staudigel et al., 2006). However, the relationship between seafloor age and animal community composition was not determined owing to a lack of relevant time series datasets from Nafanua and a lack of age constraints.
The age, location, extent, and size of eruptive events can be quantified through georeferenced calculations of the area and volume of lava flows derived from time-series bathymetry datasets. Here, we re-examine and quantify the bathymetric changes between 1999-2005 and identify additional depth changes between subsequent bathymetric surveys at Vailulu’u in September 2012 by the R/V Revelle (RR1212, EM122) and in February 2017 by the NOAA ship Okeanos Explorer (EX1702, EM302). Through a differential analysis of this time-series bathymetry dataset, spanning from 1999 to 2017, we assign maximum age ranges to the different portions of Nafanua. We relate high-definition ROV imagery collected in 2017 with the assigned seafloor ages to describe the early stages of colonization and primary ecological succession in the non-vent benthic ecosystems on Nafanua.
Materials and methods
Bathymetry analyses
We used MB-System software v5.6.20181016 (Caress and Chayes, 2016) to compare the bathymetric data collected in 1999, 2005, 2012, and 2017. First, we gridded each dataset with a 30-m grid cell size (Figures 2A–D). We then subtracted one grid from another to determine depth changes (Figures 2E–H). We then calculated the area and volume of significant depth changes using ArcGIS software (ESRI, Redlands, CA). See the Supporting Information file for thickness, area, and volume data. Not all the areas of apparent depth change are real because false changes can be created due to noise in the sonar data or lower-quality ship navigation in individual surveys, especially in areas with steep slopes. Generally, a depth-change threshold is used to distinguish signal from noise. Above this threshold, depth changes are deemed significant if they can be confirmed by ground-truth observations from submersibles and are geologically reasonable (Chadwick et al., 2019).
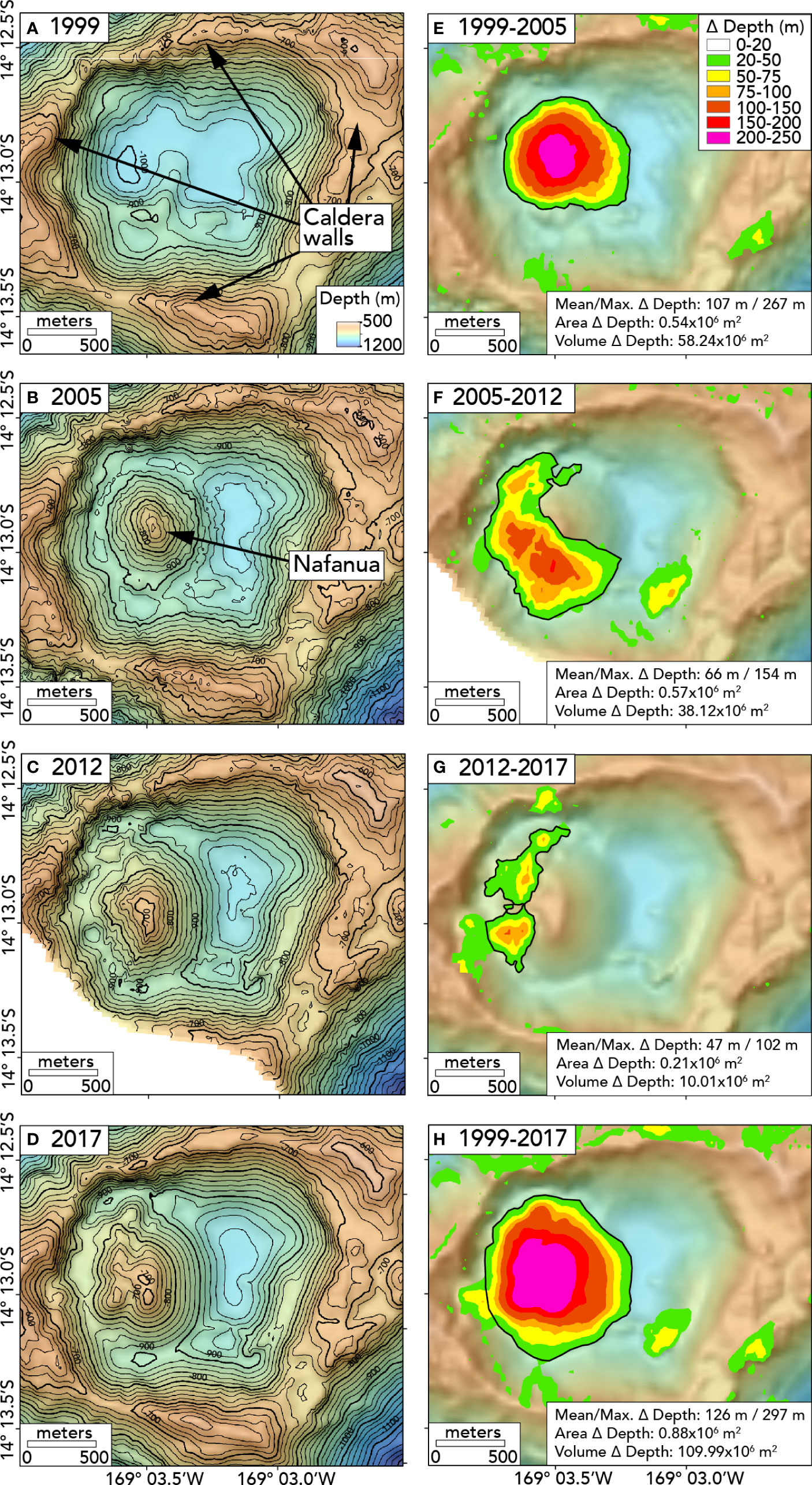
Figure 2 Maps showing bathymetry in the caldera of Vailulu’u Seamount in different years on left: (A) 1999, (B) 2005, (C) 2012, (D) 2017, and the depth differences between the surveys: (E) 1999-2005, (F) 2005-2012, (G) 2012-2017, (H) 1999-2017. The black lines in (A–D) show 20m depth contours. The black outlines in (E–H) show the depth changes (Δ depth) included for the area and volume calculations (additional details in Table S1). All maps are projected in UTM zone 59S.
To focus on the growth of the Nafanua cone, we ignored all negative depth changes and all changes outside the caldera. We considered zones with a positive depth difference larger than +20 m potentially significant (the brightly colored zones in Figures 2E–H), based on previous experience with bathymetric resurveys elsewhere (Chadwick et al., 2019) and the quality of the bathymetric datasets at Vailulu’u. However, even above this +20 m threshold, some zones were excluded from the area and volume calculations. These include the zones of positive change on the SE and N caldera rim in the 1999-2005 comparison (up to +57 m and +68 m, Figure 2E) and the zone of change on the west and north caldera wall (upslope of the contact between Nafanua and the wall) in the 2005-2012 and 2012-2017 comparisons (up to +68 m, Figures 2F, G). These zones were not considered because of our focus here is on Nafanua. However, a zone of positive change on the SE caldera floor in the 2005-2012 comparison (up to +95 m, Figure 2F) could represent a landslide deposit from the SE caldera wall since it appears in both the 2012 and 2017 bathymetric maps (Figures 2C, D) as well as the 2005-2012 and 1999-2017 comparisons (Figures 2F, H). Nevertheless, we exclude it from Nafanua volume calculations in Table S1 as we focus only on bathymetric changes of the cone and the emplacement of new lava flows.
ROV deployment
On February 24, 2017, a two-bodied remotely operated vehicle system (ROV Deep Discoverer –D2– and Seirios) was deployed from the NOAA ship Okeanos Explorer (Dive 9 of expedition EX1702) to survey the Nafanua cone within the Vailulu’u caldera. D2 was equipped with a Sea-Bird Scientific (Bellevue, WA, USA) conductivity-temperature-depth (CTD) instrument outfitted with dissolved oxygen and turbidity sensors. The location of D2 was tracked in real-time using an ultra-short baseline (USBL) acoustic positioning system. D2 was also equipped with an Insite Pacific (San Diego, CA, USA) Zeus Plus high-definition camera and paired lasers separated by 10 cm for size scale. Close-up imaging was performed on terrain and individual animals at the discretion of the lead scientists. The entire dive was recorded (ProRes 1080p, five megabits per second) and split into 5-minute video segments.
The ROV dive began by descending to the caldera floor north of Nafanua. However, the water was highly turbid there—likely owing to hydrothermal venting (Staudigel et al., 2006)— and the ROV pilots could not operate in such limited visibility. The ROV relocated from the caldera floor to the shallower NE summit of Nafanua (not in view of the seafloor during the transit), returned to the seafloor, and then traversed in a counterclockwise direction between the four summit zones of the cone during the rest of the dive (Figure 3). Imaging surveys presented here are limited to this summit traverse over the four summit zones, spanning a depth range between 675 and 734 meters (Table S2). The linear distance of the ROV survey was estimated using the measure tool in QGIS v3.26 (QGIS Development Team 2019). The area of the surveyed seafloor was estimated as a product of linear distance and the mean image field width.
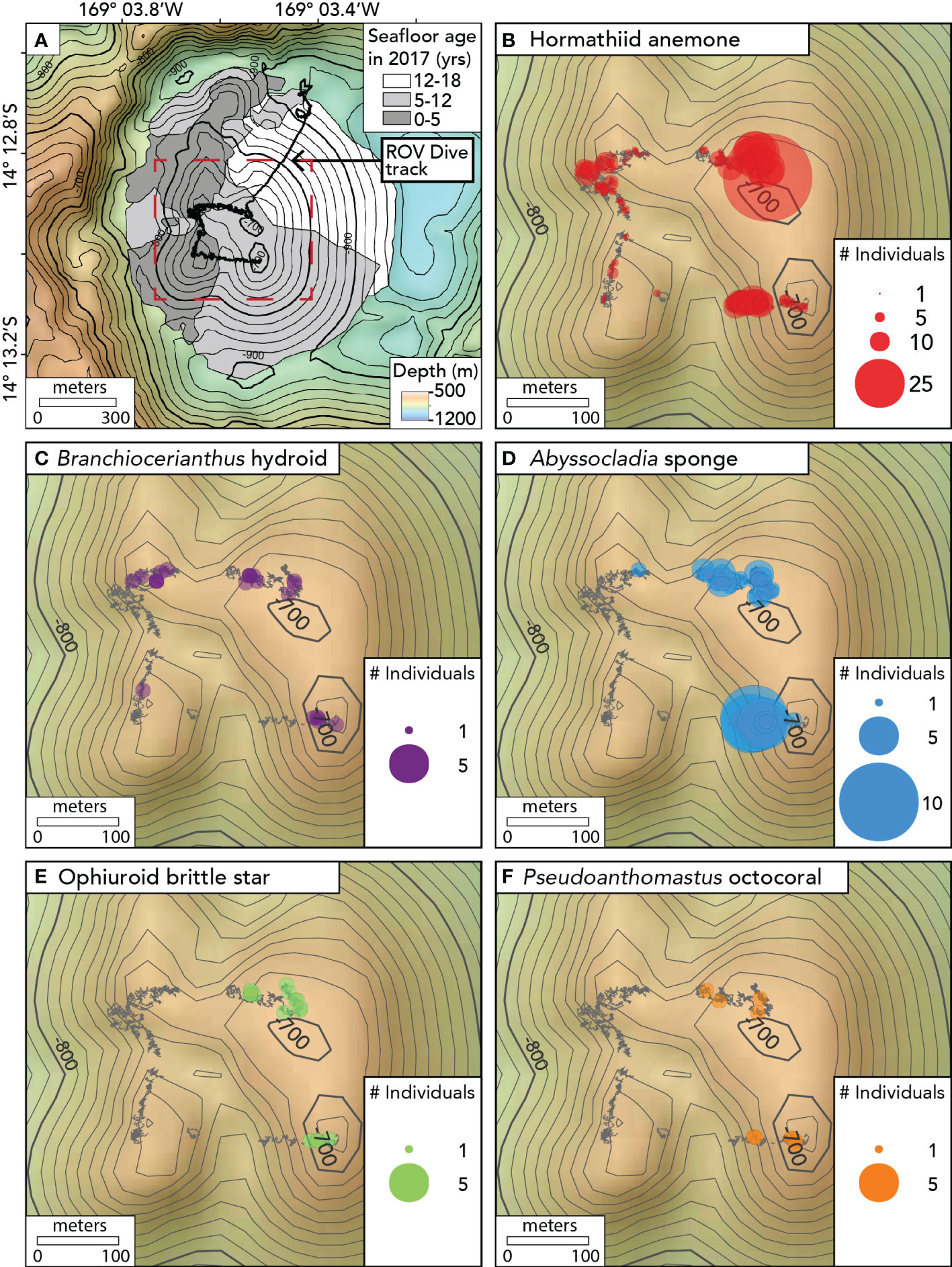
Figure 3 Maps showing the seafloor age and the distribution of benthic animal observations on Nafanua cone in Vailulu’u caldera in 2017. (A) Seafloor age ranges based on the depth changes between multibeam surveys, with the track of ROV D2 dive 9 of the expedition EX1702 overlain. Age ranges are indicated with gray areas. The black depth contours are separated by 20m. The seafloor age areas on Nafanua in this figure are the same as the areas outlined in bold in Figures 1F, G, and H, and The red dotted box indicates the location of the area where ROV benthic surveys were conducted and defines the extent of the maps shown in Figures 3B–F. (B) Hormathiid anemone observations. (C) Branchiocerianthus hydroid observations. (D) Abyssocladia sponge. (E) Ophiuroid brittle star. (F) Pseudoanthomastus octocoral. Individual animal observations within 1 meter of each other were binned together using the Point Cluster symbology (QGIS). The points’ radius is proportional to the number of observations in each 1-meter bin. The gray ‘fuzzy’ lines indicate the D2 ROV track when the vehicle was within 5 meters of the seafloor. The black depth contours are separated by 10m.
We estimated the area of the surveyed seafloor by sampling one frame per minute of video using the Python library OpenCV v4.6.0.66. The width of imaged seafloor was only calculated from frames taken when 1) the ROV was transecting, 2) the scale lasers were on, 3) and the camera was fully zoomed out. In total, 99 frames met these criteria. The image field width in each of these frames was determined in ImageJ using the known distance between scale lasers. The mean image field width was multiplied by the linear distance surveyed in each seafloor region.
Video analyses
Video analysis was conducted to characterize the biological assemblages inhabiting older (5-12 yrs) and younger seafloor (0-5 yrs) on the Nafanua cone, where ages were determined using differential bathymetry (see Figures 2, 3). The occurrences of all organisms in the video were annotated independently in triplicate (3 separate observers) using the Video Annotation and Reference System–VARS v8.3.4 (Schlining and Stout, 2006). Annotations were conducted on the same high-resolution monitor to ensure consistency. Organisms were identified to the lowest possible taxonomic level. Annotation data were merged by time with navigation and environmental data. We limited the dataset to include only annotations of organisms when the bottom was clearly in view. The analyses did not incorporate instances when the ROV was at an altitude greater than 5 m above the seafloor because we could not confidently perform animal identifications above that threshold.
We focused the biological video analysis on observed, low-mobility animals (sessile and sedentary): a morphospecies of anemone (Family: Hormathiidae), the carnivorous sponge Abyssocladia cf. brunni (Family: Cladorhizidae), the hydroid Branchiocerianthus sp. (Family: Corymorphidae), a morphospecies of brittle star (Order: Ophiurida), and the mushroom octocoral Pseudoanthomastus sp. (Family: Coralliidae). Absolute morphospecies abundance was counted for each replicate set of annotations and averaged. We performed a two-sided Fisher’s exact test of independence in R v4.1.2 to test for differences in the relative abundances of observed morphospecies between older (5-12 yrs) and younger seafloor (0-5 yrs) on the Nafanua cone. The Bray–Curtis dissimilarity index (Bray and Curtis, 1957) was also calculated to quantify the community composition differences between the two sites using the R package vegan v2.6-2 (Oksanen et al., 2007). The Shannon and Simpson α-diversity indices (Shannon, 1948; Simpson, 1949), and Pielou’s evenness (Pielou, 1966), were also calculated using vegan.
Anemone size analysis
Diameters of the oral discs of observed anemones were measured opportunistically whenever the following criteria were met: 1) the laser scale was visible in the same plane as the anemone, 2) the anemone was oriented towards the camera with extended tentacles, and 3) the anemone was imaged during a close-up. Anemones were measured by taking 1920 x 1080 pixel frame grabs, and the diameter of the oral disc was measured in ImageJ using the distance between lasers to set the scale.
Results
Bathymetric changes
After the Nafanua cone was initially formed between 1999-2005, most of the growth occurred on the western and southwestern sides of the cone. Seafloor depth changes between 2005-2012 and 2012-2017 indicate that lava flows were emplaced predominantly in the zone between the original summit of the cone and the western caldera wall (Figure 2, Table S1, see Supporting Information for details). The 2017 bathymetry shows a second ridge of lava west of the original summit of Nafanua. These observations suggest that the eruptive vents for the new lava migrated slightly westward with time.
The volume change between 1999-2005, 58.2 x 106 m3, was the largest of any time interval, and the change in volume with each subsequent comparison decreased with time (Table S1). Likewise, the average eruption rate over each time interval decreased from 9.7 x 106 m3/yr (1999 to 2005) to 5.4 x 106 m3/yr (2005 to 2012) and then to 2.0 x 106 m3/yr (2012 to 2017). The average annual volume change over the entire study period (i.e., 1999 to 2017) was 6.5 x 106 m3/yr. The maximum depth change within each time interval was greater than 100 m, but the depth change was the largest (267 m) between 1999-2005, when the Nafanua cone first formed as a new feature in the caldera floor. The net changes over the entire time interval (1999-2017) are 297 m in thickness, 0.875 x 106 m2 in area, and 110.0 x 106 m3 in volume (0.11 km3).
Using differential bathymetry collected across the 3 time intervals—1999 to 2005, 2005-2012, and 2012-2017—we constrained the age of the seafloor (relative to 2017) to interpret the patterns of biological colonization and community succession on Nafanua. In the area where the depth changes were constrained between 2012-2017, the seafloor was 0-5 years old, the area of 2005-2012 depth changes was 5-12 years old, and the area of 1999-2005 changes was 12-18 years old (Figure 3A).
ROV deployment
We traversed between the four summits of the Nafanua cone during an ROV Deep Discoverer dive in 2017, covering an estimated distance of 610 m (average speed 0.06 knots) (Figure 3). The survey distance over the older seafloor zone (5-12 years old) was 230 m, compared to 280 m over the younger seafloor zone on Nafanua (0-5 years old). The estimated survey area in the older seafloor zone was approximately 1,188 ± 97 m2 (mean ± 1.96 standard error), and 1,190 ± 118 m2 in the younger seafloor zone (Table S2). The environmental conditions and visibility encountered in both zones were comparable. The oldest seafloor zone (12-18 years old) on Nafanua was not directly observed during the dive due to poor visibility and an off-bottom transit. The 5-12 year-old seafloor had brown-colored rocks that were more altered and covered with pyroclastic sediments than the 0-5 year-old seafloor. The younger seafloor zone revealed exceptionally fresh lava surfaces consistent with their more recent eruption age.
Biological assemblages
In total, 1,552 individuals of five sessile or sedentary morphospecies were observed during the ROV dive. The most abundant morphospecies was a hormathiid anemone, with 1,338 individual observations, followed by the carnivorous chladorizhid sponge Abyssocladia cf. brunni, with 163 individuals. Brachiocerianthus sp. solitary hydroids, a brittle star morphospecies, and Pseudoanthomastus sp. mushroom octocorals numbered less than 25 individuals each.
Animal assemblages differed in abundance, density, and composition between the older and younger seafloor zones (Figures 3, 4, Tables S3, S4). We observed an overall greater abundance and density of organisms on the 5-12 year-old seafloor (534 animals per 100 m of survey; 0.86 ± 0.07 animals per m2 [mean ± 1.96 standard error]) than on the 0-5 year-old seafloor (191 animals per 100 m of survey; 0.22 ± 0.02 animals per m2). All five animal morphospecies were observed in the 5-12 year-old seafloor zone, whereas the Pseudoanthomastus sp. octocoral and the brittle star morphospecies were absent from the 0-5 year-old seafloor zone (Figure 3). Differences in morphospecies abundances were statistically significant between seafloor age zones (Fisher’s exact test, p < 0.001). Consistently, the Bray-Curtis dissimilarity index between seafloor zones was 0.58 (a value of 0 indicates identical sites, and a value of 1 indicates completely different sites). Shannon and Simpson indices of α diversity (indicative of the species diversity within an area) and Pielou’s evenness index (indicative of how similar the abundances of different species in a community are) were all higher in the older seafloor zone.
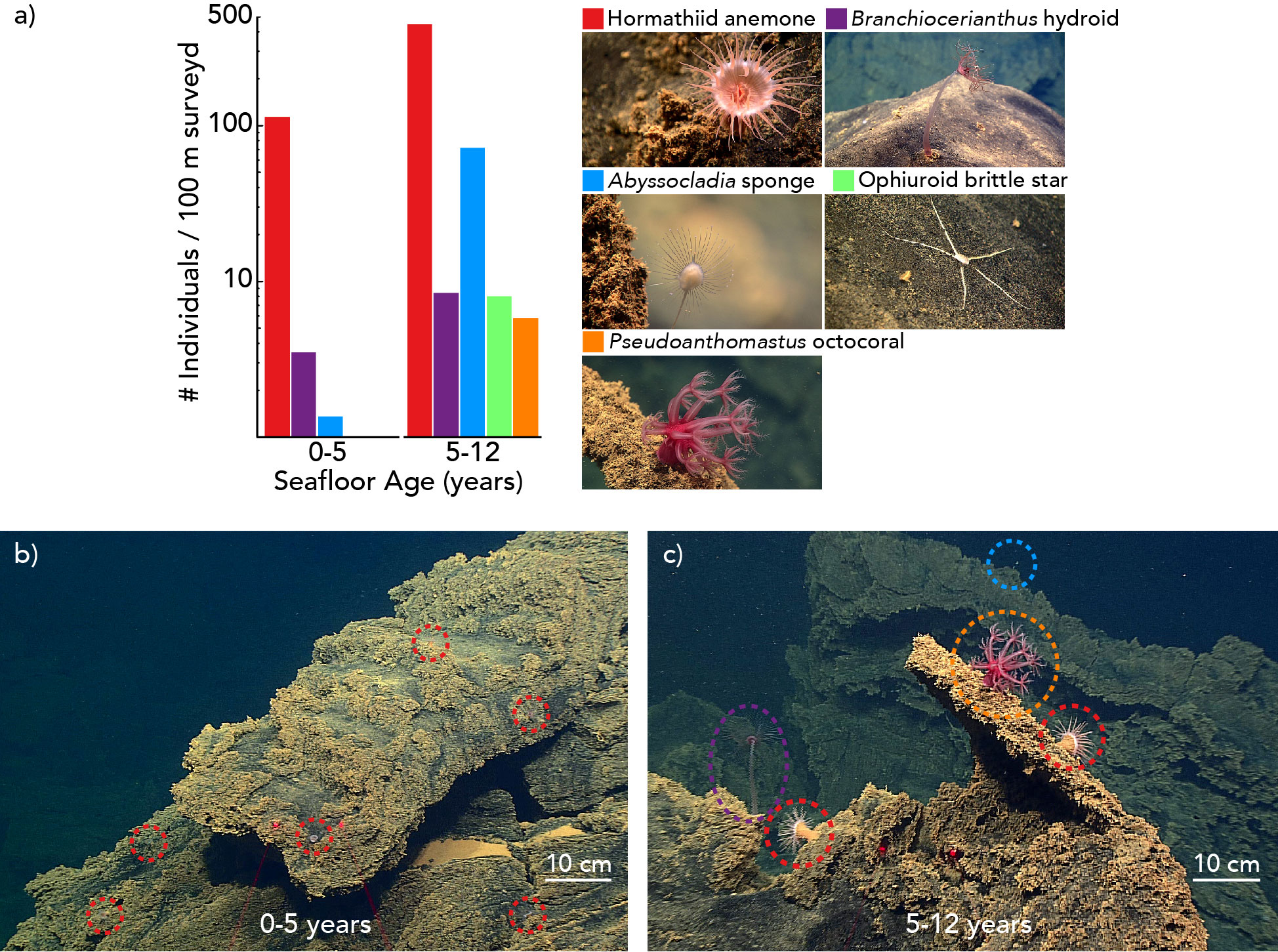
Figure 4 Benthic biologic communities in 0-5 and 5-12 year-old seafloor habitats on the Nafanua cone of Vailulu’u seamount observed during ROV D2 dive 9 of expedition EX1702 in 2017. Colors indicate different morphospecies. (A) Animal abundances by morphospecies as the number of individual animals observed per 100 meters of seafloor surveyed (linear distance). The y-axis is log10 transformed. (B) Representative image of the 0-5 year-old ecosystem. (C) Representative image of the 5-12 year-old ecosystem.
In 2005, many individuals of the synaphobranchid eel Dysommina rugosa were reported living in a diffuse venting site dubbed ‘Eel City’, located on the then summit of Nafanua (Staudigel et al., 2006). In 2017, we re-visited the approximate location of the ‘Eel City’, but did not observe low-temperature diffuse venting or eel aggregations. Inaccurate navigation data could explain this finding, or it may suggest that the site was either paved over by lava by 2017 or became extinct when fluid flow ceased. A few solitary synaphobranchid eels were observed throughout the ROV dive in 2017.
Animal sizes
Animal size measurements were performed for the anemone morphospecies observed on Nafanua. The largest anemones were observed on the 5-12 year-old seafloor zone (maximum oral disc diameter 2.42 cm versus 1.47 cm in the older zone) (Table S5). The criteria established to measure the oral disc diameter of anemones were met in only 23 instances (see Methods). Of these 23 anemones, only 3 were from the 0-5 year-old seafloor zone, preventing any meaningful statistical analysis. Both a greater number of camera close-ups performed during the survey of the younger zone of Nafanua (52 versus 30 in the older zone) and the difference in abundance between the two zones may have contributed to this discrepancy in sample size. The sizes of Branchiocerianthus sp. hydroids were also seemingly larger in the older zone than in the younger zone. However, no quantitative measurements were possible because the laser scales were not consistently used when these animals were observed.
Discussion
Eruptive dynamics
The evidence of hydrothermal activity (Hart et al., 2000), followed by the discovery of ongoing eruptive activity, fundamentally changes our view of Vailulu’u. The new analysis of repeated bathymetric surveys presented here shows that the seamount experienced multiple intermittent eruptive episodes, or perhaps semi-continuous eruptive activity, over a ~20-year period. Such long-term semi-continuous eruptive activity is rare at submarine volcanoes (Rubin et al., 2012). On the other hand, submarine volcanoes that are active for long periods are more likely to be encountered by exploratory oceanographic expeditions. For example, the only visual observations of deep-sea eruptive activity have been made at NW Rota-1 and W Mata volcanoes in the Mariana arc and the NE Lau Basin, which were active for multiple years at a time, as documented by repeated bathymetric surveys and ROV dives (Embley et al., 2006; Chadwick et al., 2008a; Clague et al., 2011; Resing et al., 2011; Chadwick et al., 2012; Schnur et al., 2017; Chadwick et al., 2019). Monowai, in the Kermadec Arc, is another seamount that has experienced multiple historical eruptions detected hydroacoustically and documented by repeat bathymetry (Chadwick et al., 2008b; Wright et al., 2008; Watts et al., 2012). The most dramatic recent example of a multi-year submarine eruption is offshore the island of Mayotte, in the North Mozambique channel, which is the largest ever documented (Lemoine et al., 2020; Berthod et al., 2021; Feuillet et al., 2021).
There are tens of thousands of seamounts in the Ocean (Kim and Wessel, 2011), and most begin as active volcanoes like Vailulu’u. Like Samoa, volcanic hotspots in the Pacific are often anchored by young and active volcanoes. For example, the Lō‘ihi seamount is found at the Hawai’i hotspot (Malahoff et al., 1982; Moore et al., 1982), the Macdonald seamount is at the Cook-Austral volcanic lineament (Stoffers et al., 1989), young seamounts are associated with the Pitcairn hotspot (Devey et al., 2003; Hekinian et al., 2003), and young seamounts are at the Societies hotspot (Talandier and Kuster, 1976; Devey et al., 2003). Thus, the eruptive evolution of Vailulu’u seamount and the ecological succession dynamic of its communities may be relevant for the inception of ecosystem development at other volcanic hotspots, as well as a significant fraction of seamount ecosystems that develop in other volcanic settings (i.e., subduction and mid-ocean ridge volcanism) globally. Being able to document the early stages of ecological succession between dated eruptive episodes, as we have done at Vailulu’u, is a rare and unique scientific opportunity.
Successional model
Our observations of the animal community structure on Nafanua indicate a pattern of early primary succession. Consistent with established ecological theory, the abundance, sizes of organisms, and species diversity on Nafanua increased with the age progression of the seafloor habitat. The changes in species composition over time at Vailulu’u suggest a sequence where the colonization of new seafloor is started by pioneer species, followed by the arrival of later successional species (Figure 5).
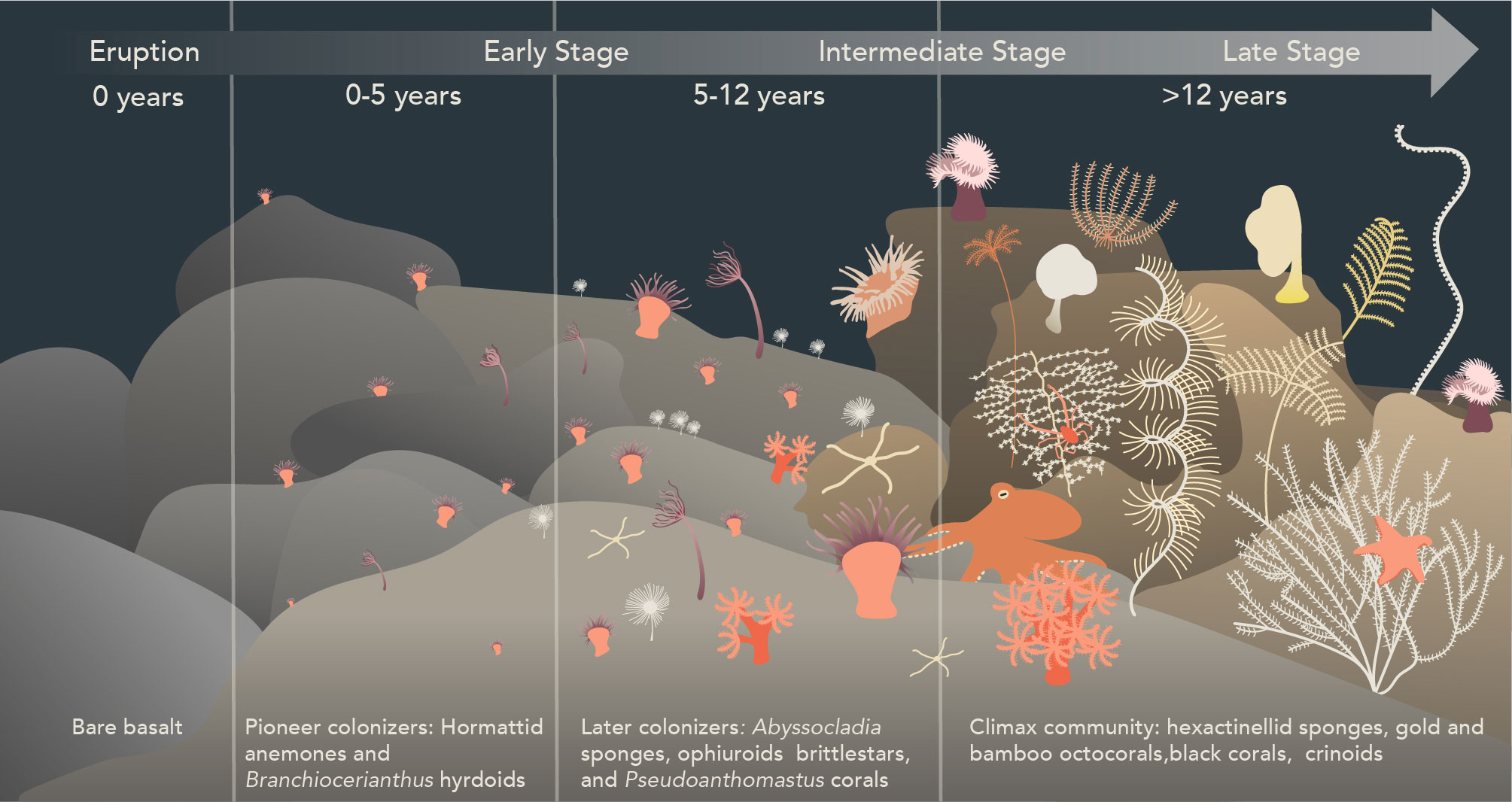
Figure 5 Inferred post-eruption ecological succession stages in non-vent communities on Vailulu’u seamount, American Samoa. New seafloor is first colonized by pioneer species of hormathiid anemones and Branchiocerianthus hydroids, followed by Pseudoanthomastus sp. octocorals, Abyssocladia cf. brunni sponges, and free-living ophiuroids. Later successional stages are likely characterized by the replacement of anemones and hydroids by slow-growing and long-lived species such as bamboo and gold octocorals, antipatharian black corals, comatulid and stalked crinoids, echinoid sea stars, and hexactinellid glass sponges. This figure was hand-drawn using Adobe Illustrator 2023.
The hormathiid anemone and Branchiocerianthus hydroid species observed in 2017 on Nafanua are likely examples of pioneer species that colonize recently erupted areas. Pioneer species in terrestrial ecosystems are typically r-selected species characterized by small size, rapid growth, high fecundity, high dispersive potential, and short life spans (Huston and Smith, 1987). Such r-selected life history characteristics have been documented in another deep-sea aplanulate hydroid species, Bouillonia cornucopia, consistent with the idea that some hydroids may be pioneer species in the deep sea (Meyer-Kaiser et al., 2021). Hydrozoan and bryozoan small-size species capable of colonizing bare surfaces have also been documented in higher frequency in disturbed seamounts relative to undisturbed ones. They thus are presumed to be early colonizers species (Clark and Rowden, 2009). The anemone and hydroid pioneer species observed on Vailulu’u in 2017 were not observed in 2005 (Staudigel et al., 2006). Their absence from surveys of Nafanua in 2005 could be attributed to a short time since eruption relative to the timing of the submersible Pisces V dives in late March of 2005. A piece of Nafanua basalt sampled by dredging in 2005 was constrained with 210Po dating to have erupted on November 8, 2004 (+62/-67 days, 2 sigma) (Sims et al., 2008). This basalt age indicates that Nafanua erupted as little as 3 to 5 months before the Pisces V surveys, apparently leaving too little time to observe the colonization of Nafanua by pioneer species. Consistent with this idea, the presence of hydrothermal venting on the summit of Nafanua in 2005 (‘Eel City’) could be explained by residual heat from a recent eruption (Staudigel et al., 2006). Its absence in 2017 may be due to cooling of the rock over time.
Pseudoanthomastus sp. octocorals, Abyssocladia cf. brunni sponges, and free-living ophiuroids likely colonized Nafanua later in the successional sequence. Pseudoanthomastus sp. is an octocoral without a skeletal axis from the family Coralliidae (McFadden et al., 2022). The largest Pseudoanthomastus sp. colonies observed on the 5-12 year-old seafloor zone of Nafanua had measured stalk diameters of ~2cm. A growth model for Heteropolypus ritteri, a close relative to Pseudoanthomastus, indicates that this stalk diameter may correspond to an organismal age of 6 years (Cordes et al., 2001), consistent with the age of Nafanua’s older seafloor zone (5-12 years old). Other octocorals without a skeletal axis or with an axis made of sclerites have been observed as early colonizers of disturbed Pacific deep-sea habitats. Baco et al. (2019) found soft corals (probably Family Nidaliidae) on seamount areas 300 mbsl that had been disturbed by bottom trawling 30 years before. Putts et al. (2019) found precious corals (family Coralliidae) off Hawaii, at 400-450 mbsl, on lava flows as young as 63 years old. Together, these observations suggest that octocoral species without a skeletal axis or with a scleritic axis are among the first deep-sea corals to colonize disturbed seamount areas in the upper bathyal depth zone (200 to 1000 mbsl) in the Central Pacific region.
The arrival of species with low-frequency spawning and recruitment likely characterizes later successional stages. The absence of the anemone and hydroid species from the much older (pre-1999) caldera walls of Vailulu’u during the 2005 surveys may indicate that these pioneering species were replaced by other coral and sponge species (the caldera walls or rim were not surveyed in 2017). Pioneer species could facilitate the larval recruitment of intermediate and late succession species by altering local hydrodynamic processes and providing chemical cues (Gili and Coma, 1998; Privitera-Johnson et al., 2015; Slattery and Lesser, 2021). Reproductive traits are unknown for most deep-sea species. Thus, growth rate and longevity are assumed as proxies, where longevity may indicate less frequent spawning events, and slow growth may indicate a late reproductive age and reduced recruitment success due to early-life vulnerability. Thus, we expect species in late successional stages to be slow-growing and long-lived. Deep-sea corals with solid, non-scleritic skeletal axes (e.g., isidid bamboo octocorals, chrysogorgiid gold octocorals, antipatharian black corals) generally grow slower and can live for centuries or millennia (Roark et al., 2006; Andrews et al., 2009; Roark et al., 2009; Prouty et al., 2016). Some deep-sea hexactinellid glass sponges are among the longest-living organisms on the planet, potentially reaching ages >10,000 years (Jochum et al., 2012). Gold octocorals (Iridogorgia sp., Metallogorgia sp., and Chrysogorgia sp.), bamboo octocorals (Lepidisis sp. and Keratosis sp.), black corals (Bathypathes sp. and Umbellapathes sp.), and glass sponges were observed on the caldera walls and the outside slopes of Vailulu’u in 2005 (Staudigel et al., 2006; Hourigan et al., 2015), as well as on older neighboring seamounts in the American Samoa region during the EX1702 expedition in 2017 (e.g., Moki Seamount). Our observations are consistent with those of Baco et al. (2019) and Putts et al. (2019), who found bamboo octocorals, gold octocorals, and black corals in seafloor zones decades to centuries post-disturbance. Thus, these deep-sea coral species, glass sponges, and other sessile species, such as stalked crinoids, may indicate late succession stages or even climax communities (i.e., the stable theoretic endpoint of ecological succession).
We present evidence indicating that deep-sea seafloor colonization by sessile species can occur within five years of its formation. The new seafloor colonization rate likely depends on the growth rate of animals, which in turn is limited by the rate of food supply (particulate organic carbon, or POC, flux). The POC flux decreases with depth and depends on the characteristics of the surface primary productivity (Martin et al., 1987; Buesseler, 1998). The hydrothermal venting from Vailulu’u could directly inject nutrients into the overlying surface waters through buoyant plumes or indirectly through vertically migrating organisms. These nutrient injections could result in a localized increase in surface primary productivity and POC flux to the deep sea, thus promoting more rapid animal growth on Vailulu’u than on neighboring inactive seamounts. Chemosynthetic primary production supported by hydrothermal venting could also provide food for non-chemosynthetic communities on Vailulu’u.
The rate of colonization of new habitats also depends on the rate of larval supply. Larval supply rates can vary as a function of the proximity and abundance of source populations and can be influenced by hydrodynamics. As mentioned above, there are no records of Nafanua’s anemone and hydroid morphospecies on other parts of Vailulu’u or neighboring seamounts. Established ecological theory predicts that these pioneer species could be capable of long-distance dispersal (MacArthur and Wilson, 1967; Huston and Smith, 1987). Alternatively, these species could be locally present in low abundance. Pseudoanthomastus sp. mushroom octocorals, A. cf. brunni sponges, and free-living ophiuroids were observed on Vailulu’u’s caldera walls and rim in 2005 (Staudigel et al., 2006). Thus, it is likely that these local populations are sources of larvae for the newer seafloor habitats on Nafanua. Local and regional larval pools—i.e., older seafloor habitats on Vailulu’u and neighboring seamounts—will likely be important sources of recruits for later successional stages on Nafanua.
The seafloor substrate changes over time, like the biological communities. The fresh lava surfaces found on the 0-5 year-old seafloor zone on Nafanua transitioned to more altered surfaces with a greater cover of pyroclastic sediments on the 5-12 year-old seafloor zone. Multiple experiments have shown that substrate characteristics, such as small-scale heterogeneity and roughness, can influence the recruitment success of colonists, i.e., recruitment increases with substrate complexity (Chabot and Bourget, 1988; Herbert and Hawkins, 2006; Coombes et al., 2015; Girard et al., 2016; Meyer-Kaiser et al., 2019). Thus, it is possible that changes in seamount substrate characteristics with time may influence the abundance of biological communities through successional stages.
Broader implications
Understanding the successional dynamics of natural seamount communities is essential to guide the management and of restoration post-disturbance ecosystems (Prach and Walker, 2011). Seamounts represent a significant fraction of the ocean basins, covering between 4.7% and 16.3% of the total area (Yesson et al., 2011). Although the global impact of bottom trawling on seamounts has not been fully quantified, it is undoubtedly extensive. In some regions, up to 88% of seamounts have documented trawling impacts (Williams et al., 2020). Interest in deep-sea mining for cobalt-rich crusts (Hein et al., 2013) constitutes a potential future threat to seamount communities. Knowledge of successional stages in seamount communities can be applied to identify signs and state of recovery and inform the timing and best practices for intervention. Further systematic observations and time-series monitoring are necessary to determine the generality and predictability of the patterns of early colonization and apparent succession at Vailulu’u.
Data availability statement
The datasets presented in this study can be found in online repositories. The names of the repository/repositories and accession number(s) can be found below: Video data from the 2017 ROV dive is available through the NOAA National Centers for Environmental Information, Ocean Exploration Video Portal, cruise 'Vicinity of American Samoa ROV/Mapping (EX1702)'. Multibeam data from 2017 is available at the NOAA National Centers for Environmental Information https://www.ngdc.noaa.gov/ships/okeanos_explorer/EX1702_mb.html.
Author contributions
SH, WC, MJ, and JK conceptualized the study. SH, MJ collected the data. SH, WC, JK, LM, NP, EB, and SM, analyzed the data. SH wrote the original manuscript draft, with contributions from all authors. All authors reviewed and edited the manuscript.
Acknowledgments
We dedicate this manuscript to the memory of Jasper Konter, who was involved in the early stages of this study. We thank Kelley Elliot, Meme Lobecker, the science and ROV teams, and the crew and officers for all their efforts. We thank Tina Molodtsova, Estefanía Rodríguez, and Allen Collins for their help with taxonomic identifications. We thank Talia Rodkey, Neal Hafner, and Aubrey Beck for their assistance with video annotation. This is PMEL contribution #4928. Support was provided by the University Corporation for Atmospheric Research, UCAR sub-awards no. Z17-28063 to SH and no. Z17-28065 to MJ, and the National Academies of Sciences, Engineering, and Medicine Gulf Research Program Early-Career Fellowship 2000013668 to SH.
Conflict of interest
The authors declare that the research was conducted in the absence of any commercial or financial relationships that could be construed as a potential conflict of interest.
Publisher’s note
All claims expressed in this article are solely those of the authors and do not necessarily represent those of their affiliated organizations, or those of the publisher, the editors and the reviewers. Any product that may be evaluated in this article, or claim that may be made by its manufacturer, is not guaranteed or endorsed by the publisher.
Supplementary material
The Supplementary Material for this article can be found online at: https://www.frontiersin.org/articles/10.3389/fmars.2023.1110062/full#supplementary-material
References
Althaus F., Williams A., Schlacher T. A., Kloser R. J., Green M. A., Barker B. A., et al. (2009). Impacts of bottom trawling on deep-coral ecosystems of seamounts are long-lasting. Mar. Ecol. Prog. Ser. 397, 279–294. doi: 10.3354/meps08248
Andrews A. H., Stone R. P., Lundstrom C. C., DeVogelaere A. P. (2009). Growth rate and age determination of bamboo corals from the northeastern pacific ocean using refined 210Pb dating. Mar. Ecol. Prog. Ser. 397, 173–185. doi: 10.3354/meps08193
Baco A. R., Roark E. B., Morgan N. B. (2019). Amid fields of rubble, scars, and lost gear, signs of recovery observed on seamounts on 30- to 40-year time scales. Sci. Adv. 5, eaaw4513. doi: 10.1126/sciadv.aaw4513
Berthod C., Médard E., Bachèlery P., Gurioli L., Di Muro A., Peltier A., et al. (2021). The 2018-ongoing Mayotte submarine eruption: Magma migration imaged by petrological monitoring. Earth Planet. Sci. Lett. 571, 117085. doi: 10.1016/j.epsl.2021.117085
Bray J. R., Curtis J. T. (1957). An ordination of the upland forest communities of southern Wisconsin. Ecol. Monogr. 27, 326–349. doi: 10.2307/1942268
Buesseler K. O. (1998). The decoupling of production and particulate export in the surface ocean. Global Biogeochemical Cycles 12, 297–310. doi: 10.1029/97GB03366
Caress D. W., Chayes D. N. (2016). MB-System: Open source software for the processing and display of swath mapping sonar data. Available at: http://www.mbari.org/data/mbsystem/.
Chabot R., Bourget E. (1988). Influence of substratum heterogeneity and settled barnacle density on the settlement of cypris larvae. Mar. Biol. 97, 45–56. doi: 10.1007/BF00391244
Chadwick W. W., Cashman K. V., Embley R. W., Matsumoto H., Dziak R. P., de Ronde C. E. J., et al. (2008a). Direct video and hydrophone observations of submarine explosive eruptions at NW rota-1 volcano, Mariana arc. J. Geophys. Res. 113, B08S10. doi: 10.1029/2007JB005215
Chadwick W. W., Dziak R. P., Haxel J. H., Embley R. W., Matsumoto H. (2012). Submarine landslide triggered by volcanic eruption recorded by in situ hydrophone. Geology 40, 51–54. doi: 10.1130/G32495.1
Chadwick W. W., Rubin K. H., Merle S. G., Bobbitt A. M., Kwasnitschka T., Embley R. W. (2019). Recent eruptions between 2012 and 2018 discovered at West mata submarine volcano (NE lau basin, SW pacific) and characterized by new ship, AUV, and ROV data. Front. Mar. Sci. 6. doi: 10.3389/fmars.2019.00495
Chadwick W. W., Wright I. C., Schwarz-Schampera U., Hyvernaud O., Reymond D., de Ronde C. E. J. (2008b). Cyclic eruptions and sector collapses at monowai submarine volcano, kermadec arc: 1998-2007. Geochem. Geophys. Geosyst. 9, 1–17. doi: 10.1029/2008gc002113
Clague D. A., Paduan J. B., Caress D. W., Thomas H., Chadwick W. W., Merle S. G. (2011). Volcanic morphology of West mata volcano, NE lau basin, based on high-resolution bathymetry and depth changes. Geochem. Geophys. Geosyst. 12, 1–21. doi: 10.1029/2011gc003791
Clark M. R., Bowden D. A., Rowden A. A., Stewart R. (2019). Little evidence of benthic community resilience to bottom trawling on seamounts after 15 years. Front. Mar. Sci. 6. doi: 10.3389/fmars.2019.00063
Clark M. R., Rowden A. A. (2009). Effect of deepwater trawling on the macro-invertebrate assemblages of seamounts on the chatham rise, new Zealand. Deep Sea Res. Part I 56, 1540–1554. doi: 10.1016/j.dsr.2009.04.015
Clements F. E. (1916). Plant succession: An analysis of the development of vegetation (Washington: Carnegie Institution of Washington). 242, 1–512.
Coombes M. A., La Marca E. C., Naylor L. A., Thompson R. C. (2015). Getting into the groove: Opportunities to enhance the ecological value of hard coastal infrastructure using fine-scale surface textures. Ecol. Eng. 77, 314–323. doi: 10.1016/j.ecoleng.2015.01.032
Cordes E. E., Nybakken J. W., VanDykhuizen G. (2001). Reproduction and growth of anthomastus ritteri (Octocorallia: Alcyonacea) from Monterey bay, California, USA. Mar. Biol. 138, 491–501. doi: 10.1007/s002270000470
Devey C. W., Lackschewitz K. S., Mertz D. F., Bourdon B., Cheminée J.-L., Dubois J., et al. (2003). Giving birth to hotspot volcanoes: Distribution and composition of young seamounts from the seafloor near Tahiti and Pitcairn islands. Geology 31, 395–398. doi: 10.1130/0091-7613(2003)031<0395:GBTHVD>2.0.CO;2
Dykman L. N., Beaulieu S. E., Mills S. W., Solow A. R., Mullineaux L. S. (2021). Functional traits provide new insight into recovery and succession at deep-sea hydrothermal vents. Ecology 102, e03418. doi: 10.1002/ecy.3418
Embley R. W., Chadwick W. W., Baker E. T., Butterfield D. A., Resing J. A., de Ronde C. E. J., et al. (2006). Long-term eruptive activity at a submarine arc volcano. Nature 441, 494–497. doi: 10.1038/nature04762
Feuillet N., Jorry S., Crawford W. C., Deplus C., Thinon I., Jacques E., et al. (2021). Birth of a large volcanic edifice offshore Mayotte via lithosphere-scale dyke intrusion. Nat. Geosci. 14, 787–795. doi: 10.1038/s41561-021-00809-x
Genin A. (2004). Bio-physical coupling in the formation of zooplankton and fish aggregations over abrupt topographies. J. Mar. Syst. 50, 3–20. doi: 10.1016/j.jmarsys.2003.10.008
Gili J. M., Coma R. (1998). Benthic suspension feeders: their paramount role in littoral marine food webs. Trends Ecol. Evol. 13, 316–321. doi: 10.1016/S0169-5347(98)01365-2
Girard F., Lacharité M., Metaxas A. (2016). Colonization of benthic invertebrates in a submarine canyon in the NW Atlantic. Mar. Ecol. Prog. Ser. 544, 53–64. doi: 10.3354/meps11555
Gollner S., Govenar B., Arbizu P. M., Mills S., Le Bris N., Weinbauer M., et al. (2015). Differences in recovery between deep-sea hydrothermal vent and vent-proximate communities after a volcanic eruption. Deep Sea Res. Part I. 106, 167–182. doi: 10.1016/j.dsr.2015.10.008
Grime P. J. (1979). Plant strategies, vegetation processes, and ecosystem properties (Chichester, UK: John Wiley & Sons). 222 p.
Hart S. R., Staudigel H., Koppers A. A. P., Blusztajn J., Baker E. T., Workman R., et al. (2000). Vailulu’u undersea volcano: The new Samoa. Geochem. Geophys. Geosyst. 1, 2000GC000108. doi: 10.1029/2000gc000108
Hart S. R., Staudigel H., Koppers A. A. P., Girard A. P. (2003). A fluorescein tracer release experiment in the hydrothermally active crater of vailulu’u volcano, Samoa. J. Geophys. Res. D: Atmos. 108, 1–11. doi: 10.1029/2002JB001902
Hein J. R., Mizell K., Koschinsky A., Conrad T. A. (2013). Deep-ocean mineral deposits as a source of critical metals for high- and green-technology applications: Comparison with land-based resources. Ore Geol. Rev. 51, 1–14. doi: 10.1016/j.oregeorev.2012.12.001
Hekinian R., Cheminée J. L., Dubois J., Stoffers P., Scott S., Guivel C., et al. (2003). The Pitcairn hotspot in the south pacific: distribution and composition of submarine volcanic sequences. J. Volcanol. Geotherm. Res. 121, 219–245. doi: 10.1016/S0377-0273(02)00427-4
Herbert R. J. H., Hawkins S. J. (2006). Effect of rock type on the recruitment and early mortality of the barnacle chthamalus montagui. J. Exp. Mar. Bio. Ecol. 334, 96–108. doi: 10.1016/j.jembe.2006.01.023
Hourigan T. F., Etnoyer P. J., McGuinn R. P., Whitmire C. E. (2015). An introduction to NOAA’s national database for deep-sea corals and sponges. NOAA technical memorandum NOS NCCOS. 191, 1–34. doi: 10.7289/V5/TM-NOS-NCCOS-191
Huston M., Smith T. (1987). Plant succession: Life history and competition. Am. Nat. 130, 168–198. doi: 10.1086/284704
Jochum K. P., Wang X., Vennemann T. W., Sinha B., Müller W. E. G. (2012). Siliceous deep-sea sponge monorhaphis chuni: A potential paleoclimate archive in ancient animals. Chem. Geol. 300–301, 143–151. doi: 10.1016/j.chemgeo.2012.01.009
Kim S.-S., Wessel P. (2011). New global seamount census from altimetry-derived gravity data. Geophys. J. Int. 186, 615–631. doi: 10.1111/j.1365-246X.2011.05076.x
Konter J. G., Staudigel H., Hart S. R., Shearer P. M. (2004). Seafloor seismic monitoring of an active submarine volcano: Local seismicity at vailulu’u seamount, Samoa. Geochem. Geophys. Geosyst. 5, 1–15. doi: 10.1029/2004GC000702
Lemoine A., Briole P., Bertil D., Roullé A., Foumelis M., Thinon I., et al. (2020). The 2018–2019 seismo-volcanic crisis east of Mayotte, Comoros islands: seismicity and ground deformation markers of an exceptional submarine eruption. Geophys. J. Int. 223, 22–44. doi: 10.1093/gji/ggaa273
MacArthur R. H., Wilson E. O. (1967). The theory of island biogeography. (Princeton, NJ: Princeton University Press).
Malahoff A., McMurtry G. M., Wiltshire J. C., Yeh H.-W. (1982). Geology and chemistry of hydrothermal deposits from active submarine volcano loihi, Hawaii. Nature 298, 234–239. doi: 10.1038/298234a0
Marcus J., Tunnicliffe V., Butterfield D. A. (2009). Post-eruption succession of macrofaunal communities at diffuse flow hydrothermal vents on axial volcano, Juan de fuca ridge, northeast pacific. Deep Sea Res. Part 2 Top. Stud. Oceanogr. 56, 1586–1598. doi: 10.1016/j.dsr2.2009.05.004
Martin J. H., Knauer G. A., Karl D. M., Broenkow W. W. (1987). VERTEX: carbon cycling in the northeast pacific. Deep Sea Res. A 34, 267–285. doi: 10.1016/0198-0149(87)90086-0
McFadden C. S., van Ofwegen L. P., Quattrini A. M. (2022). Revisionary systematics of octocorallia (Cnidaria: Anthozoa) guided by phylogenomics. BSSB 1, 1–79. doi: 10.18061/bssb.v1i3.8735
Meyer-Kaiser K., Bergmann M., Soltwedel T., Klages M. (2019). Recruitment of Arctic deep-sea invertebrates: Results from a long-term hard-substrate colonization experiment at the long-term ecological research observatory HAUSGARTEN. Limnol. Oceanogr. 64, 1924–1938. doi: 10.1002/lno.11160
Meyer-Kaiser K. S., Plowman C. Q., Soltwedel T. (2021). Reproduction, recruitment, and growth of the Arctic deep-sea hydroid bouillonia cornucopia. Invertebr. Biol. 140, e12332. doi: 10.1111/ivb.12332
Milligan B. N., Tunnicliffe V. (1994). Vent and nonvent faunas of cleft segment, Juan de fuca ridge, and their relations to lava age. J. Geophys. Res. 99, 4777–4786. doi: 10.1029/93JB03210
Moore J. G., Clague D. A., Normark W. R. (1982). Diverse basalt types from loihi seamount, Hawaii. Geology 10, 88–92. doi: 10.1130/0091-7613(1982)10<88:DBTFLS>2.0.CO;2
Mullineaux L. S., Adams D. K., Mills S. W., Beaulieu S. E. (2010). Larvae from afar colonize deep-sea hydrothermal vents after a catastrophic eruption. Proc. Natl. Acad. Sci. U. S. A. 107, 7829–7834. doi: 10.1073/pnas.0913187107
Odum E. P. (1969). The strategy of ecosystem development. Science 164, 262–270. doi: 10.1126/science.164.3877.262
Oksanen J., Kindt R., Legendre P., O’Hara B., Stevens M. H. H., Oksanen M. J., et al. (2007). The vegan package. Community Ecol. Package 10, 719.
Pielou E. C. (1966). The measurement of diversity in different types of biological collections. J. Theor. Biol. 13, 131–144. doi: 10.1016/0022-5193(66)90013-0
Prach K., Walker L. R. (2011). Four opportunities for studies of ecological succession. Trends Ecol. Evol. 26, 119–123. doi: 10.1016/j.tree.2010.12.007
Privitera-Johnson K., Lenz E. A., Edmunds P. J. (2015). Density-associated recruitment in octocoral communities in st. John, US virgin islands. J. Exp. Mar. Bio. Ecol. 473, 103–109. doi: 10.1016/j.jembe.2015.08.006
Prouty N. G., Fisher C. R., Demopoulos A. W. J., Druffel E. R. M. (2016). Growth rates and ages of deep-sea corals impacted by the deepwater horizon oil spill. Deep Sea Res. Part 2 Top. Stud. Oceanogr. 129, 196–212. doi: 10.1016/j.dsr2.2014.10.021
Putts M. R., Parrish F. A., Trusdell F. A., Kahng S. E. (2019). Structure and development of Hawaiian deep-water coral communities on mauna loa lava flows. Mar. Ecol. Prog. Ser. 630, 69–82. doi: 10.3354/meps13106
Resing J. A., Rubin K. H., Embley R. W., Lupton J. E., Baker E. T., Dziak R. P., et al. (2011). Active submarine eruption of boninite in the northeastern lau basin. Nat. Geosci. 4, 799–806. doi: 10.1038/ngeo1275
Roark E. B., Guilderson T. P., Dunbar R. B., Fallon S. J., Mucciarone D. A. (2009). Extreme longevity in proteinaceous deep-sea corals. Proc. Natl. Acad. Sci. U. S. A. 106, 5204–5208. doi: 10.1073/pnas.0810875106
Roark E. B., Guilderson T. P., Dunbar R. B., Ingram B. L. (2006). Radiocarbon-based ages and growth rates of Hawaiian deep-sea corals. Mar. Ecol. Prog. Ser. 327, 1–14. doi: 10.3354/meps327001
Rubin K. H., Soule S. A., Chadwick W. W., Fornari D. J., Clague D. A., Embley R. W., et al. (2012). Volcanic eruptions in the deep Sea. Oceanography 25, 142–157. doi: 10.5670/oceanog.2012.12
Schlining B. M., Stout N. J. (2006). MBARI’s video annotation and reference system. Proceedings of the Marine Technology Society/Institute of Electrical and Electronics Engineers Oceans Conference, Boston, MA, pp. 1–5.
Schnur S. R., Chadwick W. W., Embley R. W., Ferrini V. L., Ronde C. E. J., Cashman K. V., et al. (2017). A decade of volcanic construction and destruction at the summit of NW rota-1 seamount: 2004–2014. J. Geophys. Res. [Solid Earth] 122, 1558–1584. doi: 10.1002/2016JB013742
Shank T. M., Fornari D. J., Von Damm K. L., Lilley M. D., Haymon R. M., Lutz R. A. (1998). Temporal and spatial patterns of biological community development at nascent deep-sea hydrothermal vents (9°50′N, East pacific rise). Deep Sea Res. Part 2 Top. Stud. Oceanogr. 45, 465–515. doi: 10.1016/S0967-0645(97)00089-1
Shannon C. E. (1948). A mathematical theory of communication. Bell System Tech. J. 27, 379–423. doi: 10.1002/j.1538-7305.1948.tb01338.x
Sims K. W. W., Hart S. R., Reagan M. K., Blusztajn J., Staudigel H., Sohn R. A., et al. (2008). 238U-230Th-226Ra-210Pb-210Po,232Th-228Ra, and235U-231Pa constraints on the ages and petrogenesis of vailulu’u and malumalu lavas, Samoa. Geochem. Geophys. Geosyst. 9, 1–30. doi: 10.1029/2007gc001651
Slattery M., Lesser M. P. (2021). Gorgonians are foundation species on sponge-dominated mesophotic coral reefs in the Caribbean. Front. Mar. Sci. 8. doi: 10.3389/fmars.2021.654268
Staudigel H., Hart S. R., Koppers A. A. P., Constable C., Workman R., Kurz M., et al. (2004). Hydrothermal venting at vailulu’u seamount: The smoking end of the Samoan chain. Geochem. Geophys. Geosyst. 5, 1–25. doi: 10.1029/2003gc000626
Staudigel H., Hart S. R., Pile A., Bailey B. E., Baker E. T., Brooke S., et al. (2006). Vailulu’u seamount, Samoa: Life and death on an active submarine volcano. Proc. Natl. Acad. Sci. U. S. A. 103, 6448–6453. doi: 10.1073/pnas.0600830103
Stoffers P., Botz R., Cheminée J.-L., Devey C. W., Froger V., Glasby G. P., et al. (1989). Geology of macdonald seamount region, austral islands: Recent hotspot volcanism in the south pacific. Mar. Geophys. Res. 11, 101–112. doi: 10.1007/BF00285661
Talandier J., Kuster G. T. (1976). Seismicity and submarine volcanic activity in French Polynesia. J. Geophys. Res. 81, 936–948. doi: 10.1029/JB081i005p00936
Tunnicliffe V., Embley R. W., Holden J. F., Butterfield D. A., Massoth G. J., Juniper S. K. (1997). Biological colonization of new hydrothermal vents following an eruption on Juan de fuca ridge. Deep Sea Res. Part I 44, 1627–1644. doi: 10.1016/S0967-0637(97)00041-1
Waller R., Watling L., Auster P., Shank T. (2007). Anthropogenic impacts on the corner rise seamounts, north-west Atlantic ocean. J. Mar. Biol. Assoc. U. K. 87, 1075–1076. doi: 10.1017/S0025315407057785
Watts A. B., Peirce C., Grevemeyer I., Paulatto M., Stratford W., Bassett D., et al. (2012). Rapid rates of growth and collapse of monowai submarine volcano in the kermadec arc. Nat. Geosci. 5, 510–515. doi: 10.1038/ngeo1473
Williams A., Althaus F., Maguire K., Green M., Untiedt C., Alderslade P., et al. (2020). The fate of deep-Sea coral reefs on seamounts in a fishery-seascape: What are the impacts, what remains, and what is protected? Front. Mar. Sci. 7. doi: 10.3389/fmars.2020.567002
Williams A., Schlacher T. A., Rowden A. A., Althaus F., Clark M. R., Bowden D. A., et al. (2010). Seamount megabenthic assemblages fail to recover from trawling impacts. Mar. Ecol. 31, 183–199. doi: 10.1111/j.1439-0485.2010.00385.x
Wright I. C., Chadwick W. W., de Ronde C. E. J., Reymond D., Hyvernaud O., Gennerich H.-H., et al. (2008). Collapse and reconstruction of monowai submarine volcano, kermadec arc 1998-2004. J. Geophys. Res. [Solid Earth] 113, B08S03.
Keywords: colonization, ecological succession, seamount, submarine eruption, deep-sea
Citation: Herrera S, Chadwick WW Jr., Jackson MG, Konter J, McCartin L, Pittoors N, Bushta E and Merle SG (2023) From basalt to biosphere: Early non-vent community succession on the erupting Vailulu’u deep seamount. Front. Mar. Sci. 10:1110062. doi: 10.3389/fmars.2023.1110062
Received: 28 November 2022; Accepted: 04 January 2023;
Published: 19 January 2023.
Edited by:
Eleonora Puccinelli, Université de Bretagne Occidentale, FranceReviewed by:
Malcolm Ross Clark, National Institute of Water and Atmospheric Research (NIWA), New ZealandJun Ma, Institute of Oceanology, Chinese Academy of Sciences (CAS), China
Copyright © 2023 Herrera, Chadwick, Jackson, Konter, McCartin, Pittoors, Bushta and Merle. This is an open-access article distributed under the terms of the Creative Commons Attribution License (CC BY). The use, distribution or reproduction in other forums is permitted, provided the original author(s) and the copyright owner(s) are credited and that the original publication in this journal is cited, in accordance with accepted academic practice. No use, distribution or reproduction is permitted which does not comply with these terms.
*Correspondence: Santiago Herrera, santiago.herrera@lehigh.edu
†Deceased