- 1Department of General and Applied Biology, São Paulo State University (UNESP), Rio Claro, Brazil
- 2Centro de Investigación en Dinámica Celular, Universidad Autónoma del Estado de Morelos, Cuernavaca, Mexico
Health and disease emerge from intricate interactions between genotypes, phenotypes, and environmental features. The outcomes of such interactions are context-dependent, existing as a dynamic continuum ranging from benefits to damage. In host-microbial interactions, both the host and environmental conditions modulate the pathogenic potential of a microorganism. Microbial interactions are the core of the agricultural systems of ants in the subtribe Attina, which cultivate basidiomycete fungi for food. The fungiculture environment harbors a diverse microbial community, including fungi in the genus Escovopsis that has been studied as damage-causing agent. Here, we consider the ant colony as a host and investigate to what extent its health impacts the dynamics and outcomes of host-Escovopsis interactions. We found that different ant fungal cultivars vary in susceptibility to the same Escovopsis strains in plate-assays interactions. In subcolony-Escovopsis interactions, while healthy subcolonies gradually recover from infection with different concentrations of Escovopsis conidia, insecticide-treated subcolonies evidenced traits of infection and died within 7 days. The opportunistic nature of Escovopsis infections indicates that diseases in attine fungiculture are a consequence of host susceptibility, rather than the effect of a single microbial agent. By addressing the host susceptibility as a major modulator of Escovopsis pathogenesis, our findings expand the understanding of disease dynamics within attine colonies.
Introduction
Dynamics in microbial interactions derive from trade-offs between the interacting organisms, modulated by diverse environmental variables (Gonze et al., 2018). Outcomes that emerge from interacting genotypes, phenotypes, and environmental conditions, exist as a dynamic continuum (Méthot and Alizon, 2014). This includes benefits, damage, or be neutral to the interacting organisms, ultimately resulting in ecological states of mutualism, commensalism, colonization, and disease (Frey-Klett et al., 2011; Casadevall and Pirofski, 2015). Such ecological categories have no fixed boundaries, in a way that the same organism could be considered a commensal and a pathogen, depending on the ecological circumstances and other interacting species (Méthot and Alizon, 2014; Casadevall, 2017). In host-pathogen interactions, host features as susceptibility, resistance, and tolerance to infection modulate the interaction outcomes, even determining the infectivity success (Thompson, 1986; Best et al., 2009). Susceptibility refers to environmental attributes within and outside the host that favor the establishment, development, and maturation of a pathogen (Wakelin, 1978; Méthot and Alizon, 2014; Casadevall and Pirofski, 2018). These variables include host genetics, health status, and microbiome, as well as climatic conditions and chance of being infected (Casadevall and Pirofski, 2018). By mechanisms of tolerance or resistance, the host is able to limit, prevent, or reduce the probability of infection at any stage of the interaction (Wakelin, 1978; Antonovics et al., 2013; Vale et al., 2014). While a tolerant host might employ mechanisms to improve its own health to tolerate the infection (without interfering directly with the pathogen), a resistant host might directly target the pathogen and its derived toxins (Vale et al., 2014).
Cumulative evidence indicates that both the host and the ecological conditions modulate the pathogenic potential of a microorganism (Vale et al., 2014; Casadevall, 2017). Thus, the interaction dynamics determine the expression of infectivity attributes, which comprise features allowing the infection and multiplication within a host (Antonovics et al., 2013; Méthot and Alizon, 2014). Host-pathogen relationships have been historically studied through a pathogen-centric view (Casadevall and Pirofski, 1999, 2003). However, centering the pathogen as the sole disease-driving agent narrows the comprehension of complex ecological and evolutionary processes (Little et al., 2010; Méthot and Alizon, 2014). Health and disease are end results of intertwined processes within a network of players that include the host, pathogen, other members of the microbiota, and environmental properties (Restif and Koella, 2003; Lambrechts et al., 2006; Méthot and Alizon, 2014; Casadevall and Pirofski, 2015). Host-microbial interactions achieve an additional level of complexity in animal societies, where dense aggregations of individuals may favor the dispersion of both beneficial and harmful microorganisms (Biedermann and Rohlfs, 2017; Bratburd et al., 2020). Strategies to maintain and propagate beneficial microbes, as well as to avoid and inhibit the harmful ones, are thought as an important aspect of insects’ social evolution (Biedermann and Rohlfs, 2017).
Beneficial and harmful host-microbial interactions are at the core of the agricultural systems of fungus-growing ants in the subtribe Attina (Hymenoptera: Formicidae: Attina; Mueller et al., 2005; Biedermann and Rohlfs, 2017). These ants evolved an obligate mutualistic association with basidiomycete fungi cultivated in ant colonies for food (Mueller et al., 2005). Ant workers forage for leaves, seeds, insect frass and carcasses to nourish their symbiotic fungus in the fungus gardens (Martin, 1970; De Fine Licht and Boomsma, 2010). Fungal hyphae grow within the fungus garden by metabolizing the substrate, providing all nourishment for ant larvae, queen, and part of the nourishment for adult ant workers (Quinlan and Cherrett, 1979; Silva et al., 2003; Moller et al., 2011; De Fine Licht et al., 2014). The fungus garden also harbors a wide diversity of microorganisms (Fisher et al., 1996; Carreiro et al., 1997; Rodrigues et al., 2008; Aylward et al., 2012; Barcoto et al., 2020), including filamentous fungi in the genus Escovopsis (Ascomycota: Hypocreales; Currie et al., 1999a). Escovopsis was described as a specialized parasite to the ant-fungus symbiosis (Currie et al., 2003), associated with decrease in fungus garden biomass and number of workers and brood (Currie, 2001).
Patterns of phylogenetic congruence suggested a tripartite coevolution between the ants, the cultivated fungi, and Escovopsis (Currie et al., 2003; Gerardo et al., 2006a). Mechanisms of interaction (Reynolds and Currie, 2004; Folgarait et al., 2011; Marfetán et al., 2015; Varanda-Haifig et al., 2017), patterns of virulence (Currie, 2001; Wallace et al., 2014; Marfetán et al., 2015), patterns of specificity (Gerardo et al., 2004, 2006b; Taerum et al., 2007; Birnbaum and Gerardo, 2016), and detrimental impact of infection in ant colonies (Currie, 2001) have been evaluated mainly through an Escovopsis-centered perspective. Here, considering the ant colony as a host (composed by the fungus garden and the ants), we evaluate host-Escovopsis interactions in the fungiculture of higher attines. Our first approach assessed in vitro interactions between Escovopsis and the symbiotic fungi cultivated by the higher attines Atta sexdens and Mycetomoellerius tucumanus. In the second approach, we analyzed interactions between Escovopsis and queen-less colonies of A. sexdens containing ant workers, pupae, and larvae (hereafter mentioned as subcolonies). Specifically, we investigate (i) whether fungal cultivars are differentially susceptible to diverse Escovopsis strains; (ii) whether fungal cultivar-Escovopsis interactions (FEI) follow a phylogenetic distribution; (iii) how healthy subcolonies respond to infection with Escovopsis conidia in different concentrations; and (iv) how insecticide-treated subcolonies respond to Escovopsis conidia infection. Our findings evidenced the cultivar and the subcolony susceptibility as important modulators of the dynamics and outcomes of subcolony-Escovopsis interactions (SEI) in the higher attine symbiosis. When the ants and fungus-gardens are healthy, effective defenses are built preventing Escovopsis infections.
Materials and Methods
Fungal Strains
Fungal cultivar-Escovopsis interactions were examined using 21 Escovopsis strains, distributed across the phylogeny. These strains were isolated from fungus gardens of higher attine ants in the genera Atta, Acromyrmex, Mycetomoellerius, Paratrachymyrmex, and Trachymyrmex sensu lato (Table 1), and were phylogenetically analyzed by Meirelles et al. (2015). Strains were revived prior to the experiments by inoculating cryopreserved conidia on potato dextrose agar (PDA, Neogen Culture Media, Lansing, MI, United States) and incubating at 25°C for 7 days in the dark. Such conditions are reported to favor Escovopsis development (Montoya et al., 2019). The experiments included two strains of fungal cultivars: Leucoagaricus gongylophorus FF2006, obtained in 2006 from fungus gardens of an A. sexdens colony (L. gongylophorus AS) maintained at the Center for the Study of Social Insects (CEIS, UNESP); and Leucoagaricus sp. IJ2016, obtained in 2016 from fungus gardens of a M. tucumanus (Leucoagaricus sp. MT) colony collected at the UNESP campus. Strain IJ2016 was isolated on PDA supplemented with 150 μg mL–1 of chloramphenicol (Sigma, St. Louis, MO, United States). Both fungal strains are maintained in the laboratory under continuous transfer on culture medium (as described by Pagnocca et al., 1990). Cultures are considered vigorous since they fully develop staphyla and gongylidia on artificial medium.
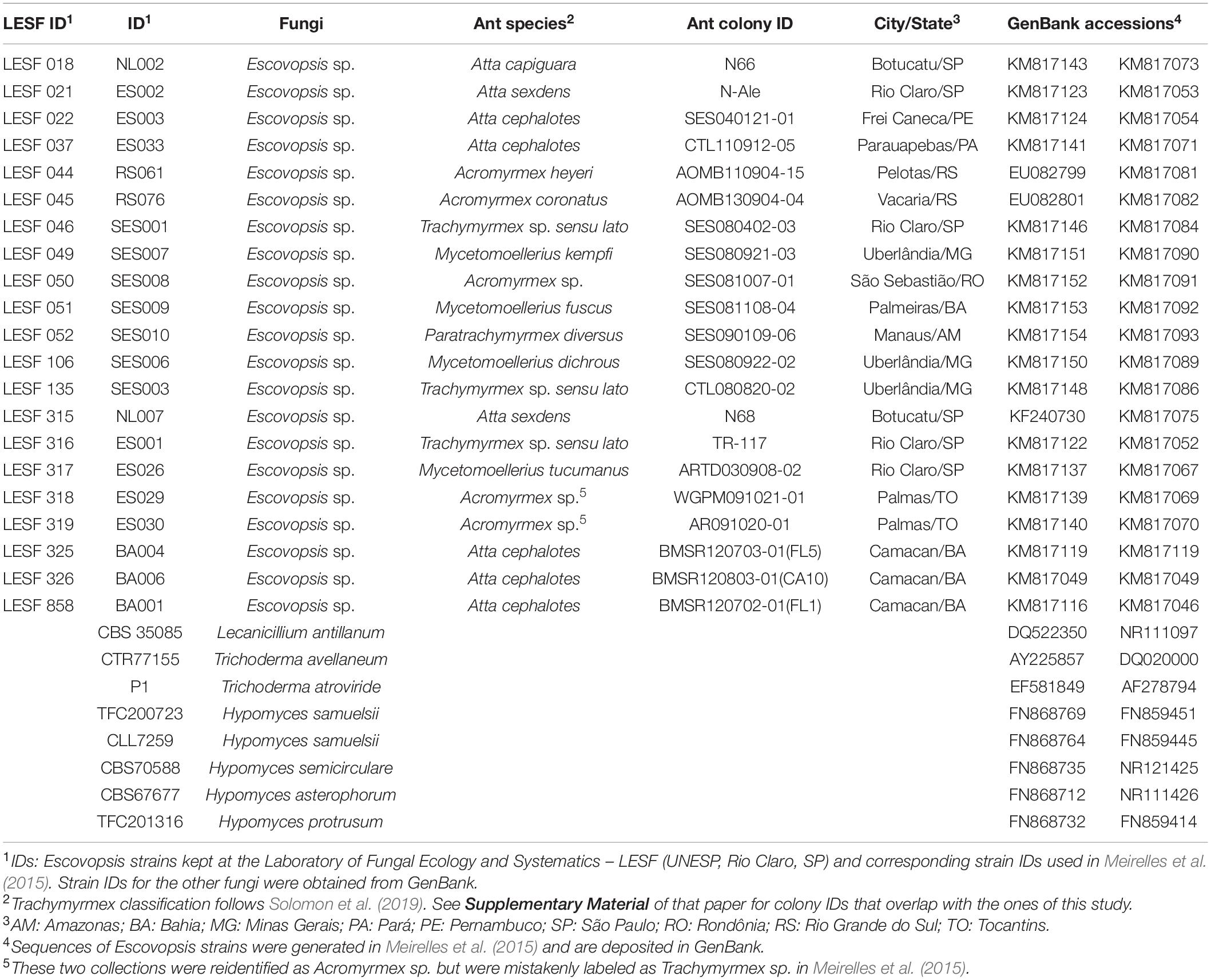
Table 1. Escovopsis strains isolated from fungus gardens of higher attine ants in Brazil and other fungal species used in the phylogenetic analyses.
Dual-Culture Assays
Fungal cultivar-Escovopsis interactions were analyzed through dual-culture assays (Silva et al., 2006; Varanda-Haifig et al., 2017). Briefly, the fungal cultivar (either L. gongylophorus AS or Leucoagaricus sp. MT) was grown for 18 days on PDA, at 25°C in the dark. From these cultures, a mycelium fragment of 0.8 cm in diameter was cut and inoculated on PDA, at 15 mm from the border of the Petri dish (Supplementary Figure 1). These plates were maintained at 25°C for 15 days in the dark. After the head-start growth of the fungal cultivar, one mycelium fragment of Escovopsis was inoculated 30 mm from the border of the ant fungal cultivar colony. Escovopsis inoculum was obtained from a culture previously grown on PDA (at 25°C for 7 days in the dark). For this experiment, two control sets of were included: (i) plates inoculated only with the fungal cultivar (either L. gongylophorus AS or Leucoagaricus sp. MT) and (ii) plates inoculated only with Escovopsis. Each tested strain and control had eight technical replicates, which were incubated at 25°C for 14 days in the dark. We used the colony growth area (in mm2) to infer the effect of Escovopsis on the fungal cultivar growth and for evaluating the Escovopsis growth rate. The growth area of the fungal cultivar was measured immediately before Escovopsis inoculation and after 1, 2, 3, 5, 7, 10, and 14 days of incubation. Colony measurements were carried out from images of digitized plates (HP Deskjet 2050-J510 scanner) in ImageJ 1.x (Schneider et al., 2012).
Fungal Cultivar and Escovopsis Growth Inhibition
Fungal cultivar-Escovopsis interactions outcomes were based on the mean values of the final area of colony growth for both fungi. Measurements were taken for all interaction plates (FEI) and for all control plates (fungal cultivar and Escovopsis growing separately). Mean values of growth areas were reported with 95% confidence intervals using a null model developed by a Monte Carlo method with 10,000 randomizations. This analysis was performed in PopTool v3.2 (p < 0.05).
For each day of experiment, growth areas in the interaction plates were standardized by the mean values reported for control plates. The mean growth area of the fungal cultivar in the presence of Escovopsis were standardized by the mean growth area values of the fungal cultivar in the control plates (Supplementary Tables 1, 2). The mean growth area of Escovopsis in the presence of fungal cultivars were standardized by the mean values of growth areas of Escovopsis in the control plates (Supplementary Tables 3, 4). Then, the standardized growth areas in the interaction plates were compared with the expected value of the control plates (area equals to 1 in a t distribution) using a t-test for single means (p < 0.05) in Statistica v8.0.360.
Fungal Cultivar-Escovopsis Interaction Patterns
Fungal cultivar-Escovopsis interactions were classified according to the mycelial growth inhibition of the fungus after 14 days of experiment. Standardized values (Supplementary Tables 1, 2) were used to estimate the fungal cultivar mycelial growth inhibition (%) by
Where:
Cultivarcontrol: Fungal cultivar mycelia area in control plates (standardized final growth area)
Cultivarinteracting: Fungal cultivar mycelia area in interaction plates (standardized final growth area).
Obtained values fell between 0% (no inhibition of fungal cultivar growth) and 100% (total inhibition of fungal cultivar growth). Growth inhibition of L. gongylophorus AS or Leucoagaricus sp. MT was compared by Mann-Whitney non-parametric test (p < 0.05) in PAST 3 (Hammer et al., 2001). Inhibition patterns were classified according to Birnbaum and Gerardo (2016) as: (i) attraction and no inhibition: Escovopsis grows directly toward the fungal cultivar, and it is not inhibited; (ii) attraction with inhibition: Escovopsis grows directly toward the fungal cultivar, but its own growth is inhibited; (iii) no attraction and no inhibition: Escovopsis neither grows directionally toward the fungal cultivar nor has its growth inhibited (Figure 1).
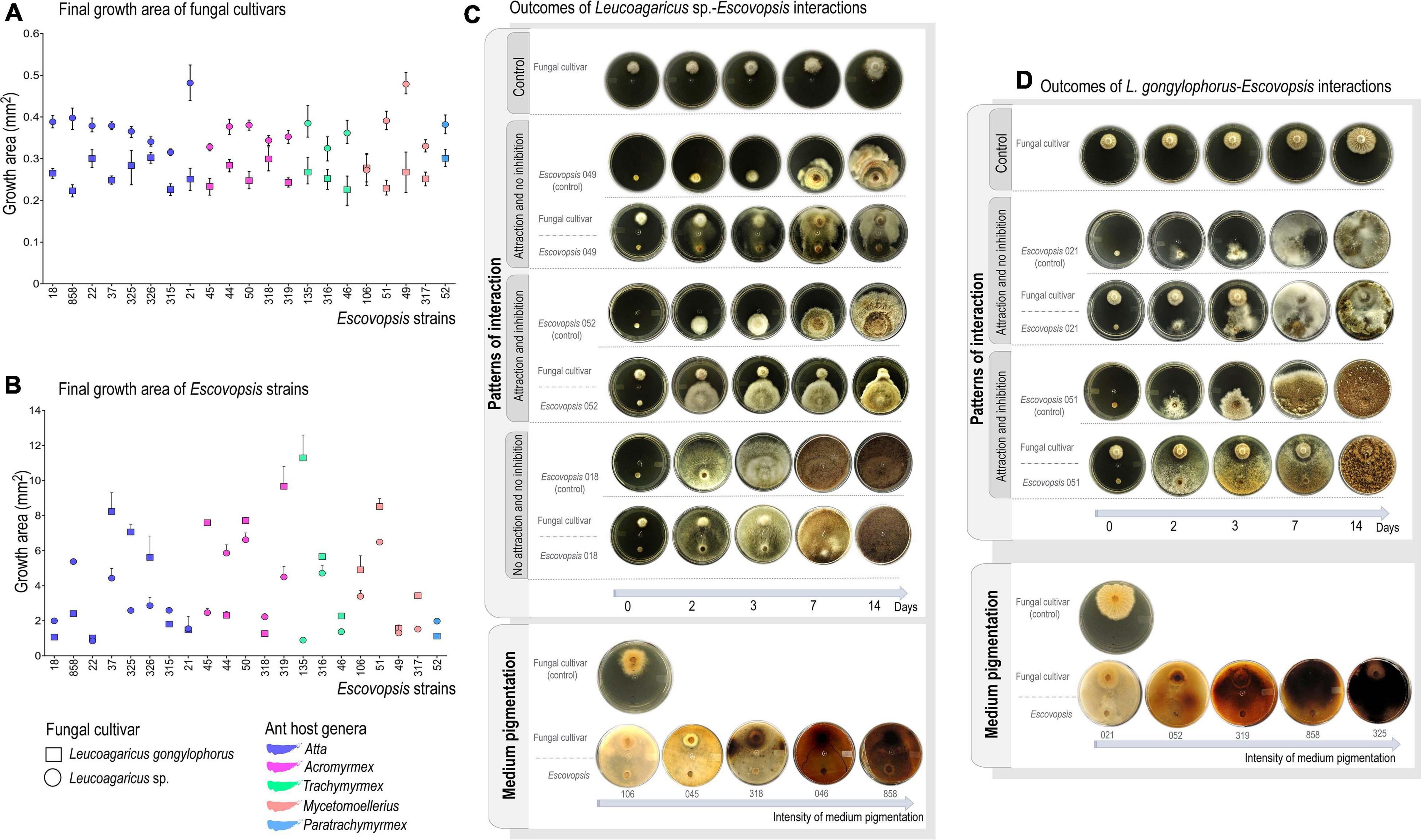
Figure 1. Interaction outcomes on plate-assays. (A) Final growth area (in mm2) of Leucoagaricus gongylophorus and Leucoagaricus sp. after interacting with Escovopsis strains for 14 days. (B) Final growth area of Escovopsis strains after interacting with fungal cultivars for 14 days. Bars correspond to 95% of confidential interval under Monte Carlo regression analysis (1,000 randomizations). (C) Classification of interactions outcomes between Leucoagaricus sp. and Escovopsis, which include the patterns of interaction observed and pigmentation of culture media. (D) Classification of interactions outcomes between L. gongylophorus and Escovopsis, including patterns of interaction and pigmentation of culture media.
Correlation Between Outcomes of Fungal Cultivar-Escovopsis Interactions and Escovopsis Phylogeny
To evaluate whether patterns of FEI have any phylogenetic underpinnings, interaction outcomes were pinpointed in the Escovopsis phylogeny. Phylogenetic analysis was performed combining 52 sequences of the internal transcriber spacer region (ITS) and of the elongation factor 1 alpha (tef1) gene. Escovopsis sequences were obtained from Meirelles et al. (2015). Sequences of two Cladobotryum, three Hypomyces and two Trichoderma strains were included as close relatives of Escovopsis and Lecanicillium antillanum was used as the outgroup (Montoya et al., 2019). GenBank accessions of the 21 strains used in the assays, seven strains used as closest relatives to Escovopsis and one strain used as outgroup are listed in Table 1. The ITS and tef1 data sets were aligned independently using MAFFT v. 7 (Katoh and Standley, 2013), edited manually, and subsequently concatenated using WINCLADA v. 1.00.08 (Nixon, 1999). The final data set contained 1,384 bp (ITS – 626 bp, and tef1 – 758 bp). Bayesian inference was applied to reconstruct the final phylogenetic tree in MrBayes v.3.2.2 (Ronquist et al., 2012), applying GTR as nucleotide substitution model for both markers independently under 95% of confidence interval of Bayesian information criterion, in jModelTest 2 (Darriba et al., 2012). Two independent runs were carried out, using three hot chains and one cold chain. A Markov Chain Monte Carlo (MCMC) sampling of two million generations was sufficient for achieving standard deviation values of split frequencies below 0.01. The first 25% of MCMC generations were discarded as “burn-in.” The final phylogenetic tree was edited in FigTree v.1.4 and Adobe Illustrator CC v.17.1.
Infection Assays
Subcolonies of A. sexdens ants, which cultivate L. gongylophorus (Weber, 1966; Mueller et al., 2017), were infected with Escovopsis conidia for assessing SEI. All subcolonies used in the infection assays were set up using workers and fungus garden sampled from healthy A. sexdens colonies (1–2 years old). The experimental system consisted of three chambers (500 mL disposable plastic containers) connected by plastic tubes: one for the foraging chamber, the central one for the fungus garden chamber, and one for the waste disposal (Supplementary Figure 2). A thin layer of Teflon® (polytetrafluoroethylene) was applied to the inner walls of the containers to prevent workers from escaping, and a layer of moistened plaster was added to the garden chamber for humidity preservation. A fragment of fungus garden (19–22 g) was carefully placed in the central chamber. Due to difficulties in determining the exact number of workers without disrupting the fungus garden structure (Currie, 2001), their number was not precisely specified. Though, fungus garden contained all worker castes (including minor, media, and major workers), eggs, larvae, and pupae in different stages of development. Subcolonies were acclimatized for 14 days before the experiment at 23–25°C, receiving Hibiscus sp. leaves ad libitum every 48 h. After acclimatized, subcolonies were randomly assigned to different treatments.
Infection of Healthy Subcolonies With Escovopsis Conidia
For the infection assay, 30 healthy colonies maintained in the laboratory were sampled to set up 90 subcolonies (Supplementary Figure 2). Escovopsis strains LESF 021, LESF 046, LESF 315, and LESF 318 were selected for this assay based on: (i) ant species from which Escovopsis strains were isolated (A. sexdens: LESF 021 and LESF 315; Acromyrmex sp. LESF 318, and Trachymyrmex sp. LESF 046; (ii) Escovopsis phylogenetic disposition of the strains: clade I (LESF 315 and LESF 046), clade II (LESF 318), and clade V (LESF 021), according to Meirelles et al. (2015); (iii) fungal cultivar growth inhibition observed in dual-culture assays; and (iv) interaction patterns observed in dual-culture assays: LESF 021 and LESF 318 (attraction and no inhibition); LESF 315 (attraction with inhibition); LESF 046 (no attraction and no inhibition).
Conidia suspensions of each Escovopsis strain were applied in four different concentrations, prepared from Escovopsis colonies grown for 7 days on PDA at 25°C in the dark. Test and control groups consisted of five randomly assigned subcolonies. Mycelial mass and conidia were suspended in 10 mL of 0.05% Tween 80. Conidia were separated from hyphal fragments in the suspension by filtering two to three times as previously described (Newmeyer, 1990; Osti and Rodrigues, 2018). The suspension was diluted to (i) 2–3 × 103 conidia mL–1, (ii) 4.3–4.6 × 104 conidia mL–1, (iii) 3.0–3.2 × 105 conidia mL–1, and (iv) 2.1–2.2 × 106 conidia mL–1, verified in a Neubauer chamber. Treatment was inoculated directly on the fungus garden surface using a hand sprayer: while test groups received 1 mL of conidia suspension, the control group received 1 mL of 0.05% Tween 80. Subcolonies were maintained in the same conditions described for acclimation for 14 days. Subcolony health conditions and foraging activity were scored daily by adapting a predefined score scale (Barcoto et al., 2017), and were statistically evaluated on each day separately using Friedman’s two-way ANOVA by ranks test in R v.3.1.0.
Infection of Insecticide-Treated Subcolonies With Escovopsis Conidia
The influence of subcolony susceptibility in SEI was evaluated by comparing the response of both healthy and insecticide-treated subcolonies to Escovopsis infection. Insecticide treatment was intended to disrupt subcolonies defenses by causing death of ant workers, through the application of 0.5 g of sulfluramid (Mirex-S®, insecticide in commercial bait) after 13 days of acclimation. For the artificial infection, LESF 046 (Trachymyrmex sp.) and LESF 318 (Acromyrmex sp.) were selected for having a slightly higher impact on healthy subcolonies in the previous assay. Conidia suspension of Escovopsis strains were applied subsequent to insecticide treatment.
The experimental setup included 48 subcolonies (assembled from 31 healthy lab colonies) assigned to six groups (each containing eight subcolonies). (i) Control: healthy subcolonies that received 1 mL of 0.05% Tween 80 solution; (ii) Escovopsis sp. LESF 318 (Acromyrmex sp.): healthy subcolonies that received 1 mL of LESF 318 conidia suspension – 7.1 × 106 conidia mL–1; (iii) Escovopsis sp. LESF 046 (Trachymyrmex sp.): healthy subcolonies that received 1 mL of LESF 046 conidia suspension – 7.3 × 106 conidia mL–1; (iv) Sulfluramid control: subcolonies treated with 0.5 g of sulfluramid that received 1 mL of 0.05% Tween 80 solution; (v) Sulfluramid + LESF 318 (Acromyrmex sp.): subcolonies treated with 0.5 g of sulfluramid that received 1 mL of LESF 318 conidia suspension – 7.1 × 106 conidia mL–1; (vi) Sulfluramid + LESF 046 (Trachymyrmex sp.): subcolonies treated with 0.5 g of sulfluramid that received 1 mL of LESF046 conidia suspension – 7.3 × 106 conidia mL–1). Conidia suspensions were prepared as described above. Subcolonies were maintained for 14 days in the same conditions set for acclimation period and scored daily (Barcoto et al., 2017). Fungi eventually overgrowing the fungus-garden were identified by morphological analysis (Montoya et al., 2019). Differences between groups were statistically evaluated on each day, using Friedman’s two-way ANOVA by ranks test in R v.3.1.0.
Results
Fungal Cultivars Are Differentially Susceptible to Escovopsis
Both fungal cultivars had their mycelial growth inhibited in the presence of all the 21 Escovopsis strains in comparison to the control (t test, p < 0.05; Figure 1A; Supplementary Tables 1, 2). FEI resulted in 51.8 to 77.7% of cultivar mycelial growth inhibition (Supplementary Tables 1, 2), resulting in death in 92.8% of interactions. Leucoagaricus sp. MT tends to be more susceptible to inhibition by Escovopsis (73.9% of mycelial growth inhibition, Supplementary Table 1) than L. gongylophorus AS (63.1% of mycelial growth inhibition; Supplementary Table 2 and Figure 1A). Such pattern is observed for both interactions between same-ant-originating cultivar-Escovopsis (for instance: L. gongylophorus AS vs. LESF 315; L. gongylophorus AS vs. LESF 021; Leucoagaricus sp. MT vs. LESF 317), and for different-ant-originating cultivar-Escovopsis (Figure 1A). Differences in cultivar susceptibility are also supported by the final growth areas of Leucoagaricus sp. MT, which were significantly lower when compared to the final growth areas of L. gongylophorus AS (Mann-Whitney, p < 0.05; Figure 1A). Differences in susceptibility are also suggested by the day when inhibitions were first detected. Leucoagaricus sp. MT was significantly inhibited by seven Escovopsis strains after the first day in dual-culture (Supplementary Table 1), while L. gongylophorus AS inhibition was observed after 2 days (Supplementary Table 2). Some FEI were characterized by accumulation of soluble pigments (Figures 1C,D). Although the three patterns of interaction described by Birnbaum and Gerardo (2016) were observed (Figures 1C,D), the predominant was attraction and no inhibition (74% of fungal symbiont-Escovopsis interactions, Figure 2).
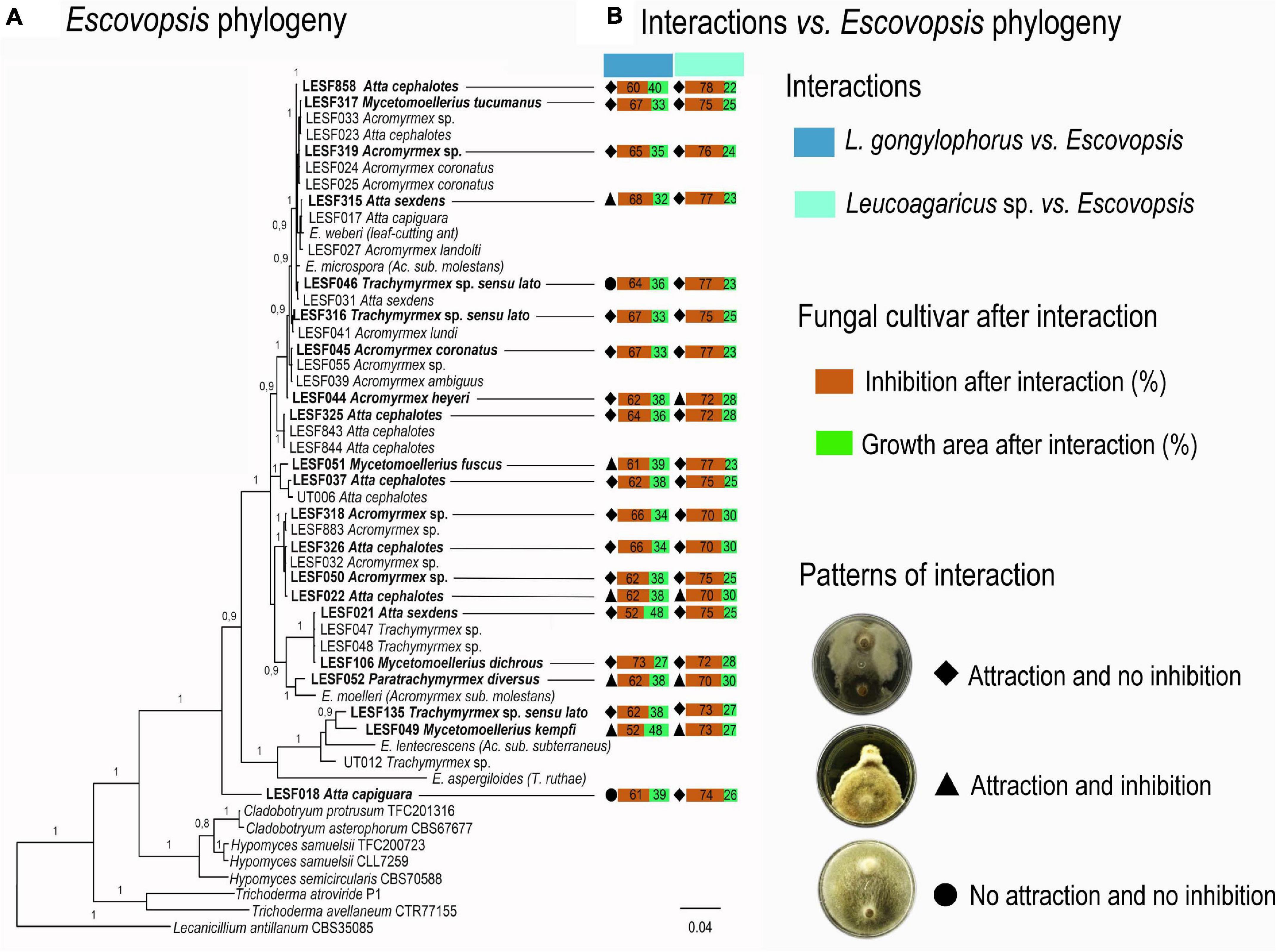
Figure 2. Phylogenetic distribution of fungal cultivar-Escovopsis interactions. (A) Escovopsis phylogeny; in bold Escovopsis strains used in the dual-culture assays. (B) Patterns of L. gongylophorus-Escovopsis and Leucoagaricus sp.-Escovopsis interactions. Growth (%) and inhibition (%) was calculated by comparing the final growth of the fungal cultivar when interacting with Escovopsis to the final growth area of the fungal cultivar growing solely. All final growth areas were standardized by the control final growth area mean values.
Escovopsis Strains Grow Faster in the Presence of Fungal Cultivars
Interaction patterns do not seem clearly delimited across Escovopsis phylogeny (Figure 2A). Leucoagaricus sp. MT was most susceptible and tended to be more inhibited by Escovopsis regardless the Escovopsis strain origin, phylogenetic distribution, and interaction patterns (Figures 1, 2). A faster growth was observed for all Escovopsis strains when interacting with both fungal cultivars (t-test, p < 0.05; Figure 1B, Supplementary Tables 3, 4). Escovopsis strains tended to grow faster when interacting with Leucoagaricus sp. MT (171%, Supplementary Table 3) than in interactions with L. gongylophorus AS (162%, Figure 1B, Supplementary Table 4). However, Escovopsis final growth areas were not directly associated with percentage of fungal cultivar inhibition (Figure 2B). For instance, Escovopsis sp. LESF 044 (Ac. heyeri) and LESF 858 (A. cephalotes) presented high final growth areas (Figure 2B), though caused low fungal cultivar inhibition (Figures 2A,B).
Healthy Fungus-Gardens Are Resistant to Infection With Escovopsis Conidia
While none of the subcolonies that received the control treatment died over 14 days of experiment, only four out of 80 (5%) subcolonies died when infected with different concentrations of Escovopsis conidia (Figure 3 and Supplementary Table 5). Of these four colonies, two were sprayed with conidia of LESF 046 (Trachymyrmex sp.) and two with LESF 318 (Acromyrmex sp.). None of the subcolonies treated with conidia of Escovopsis sp. LESF 021 (A. sexdens) and 315 (A. sexdens) died after 14 days. It is curious that even at high conidia concentration, most of the subcolonies were able to recover after 11 days of receiving the conidia treatment (Figure 3A). All Escovopsis strains significantly affected foraging activities in at least 1 day of experiment when 105 and 106 conidia mL–1 were inoculated (Figure 3B). Otherwise, none of the Escovopsis strains significantly impacted foraging activities when suspensions of 103 conidia mL–1 were applied. Overall, subcolonies treated with all conidia concentrations gradually recovered before the experiment ending (Figure 3A).
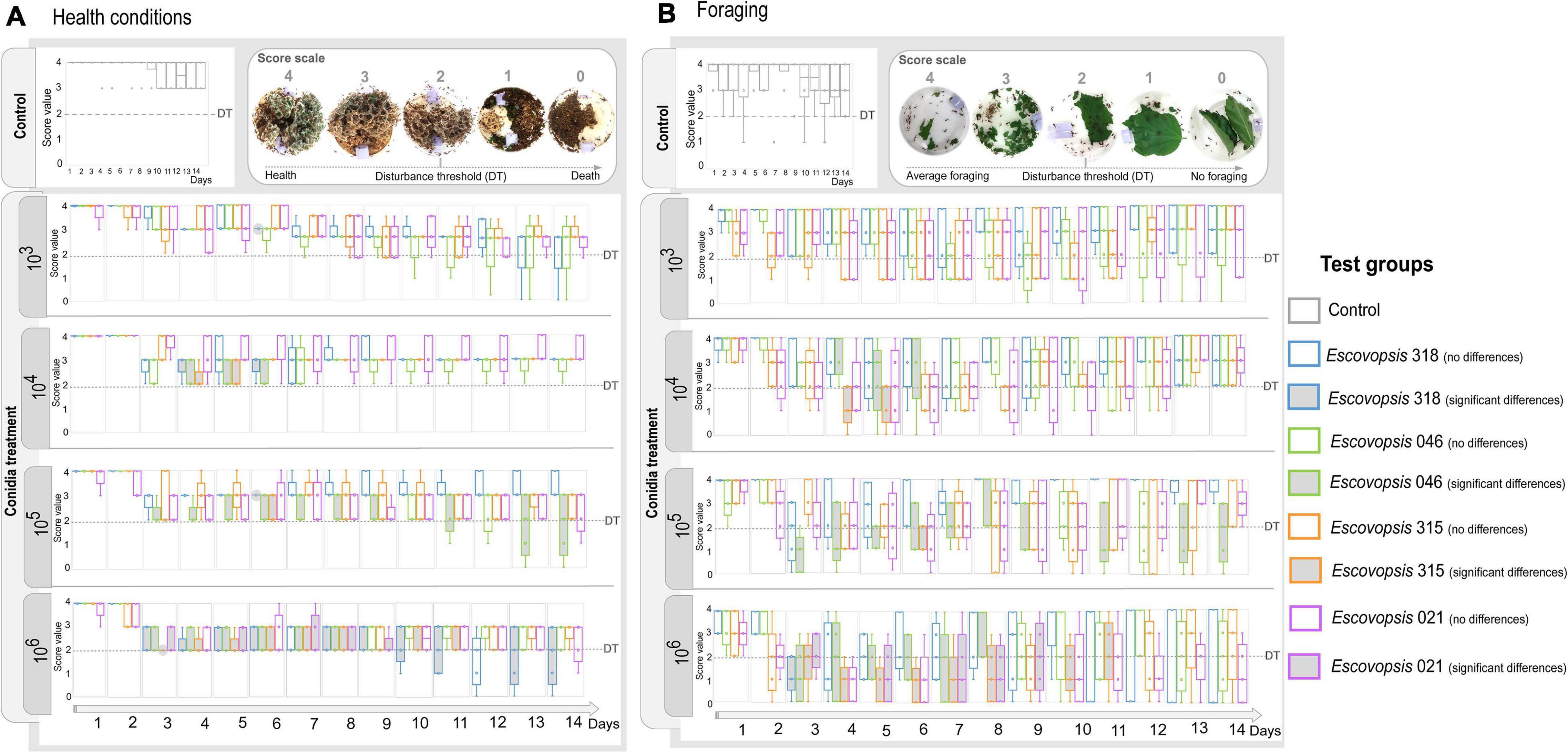
Figure 3. Changes in subcolonies followed through a score scale. (A) Health conditions and (B) foraging activity of Atta sexdens subcolonies when inoculated with different Escovopsis strains (LESF 046, LESF 318, LESF 315, and LESF 021) in different conidia concentrations (103, 104, 105, and 106 conidia mL–1), throughout 14 days of experiment. Boxes and markers in gray indicate significant differences when compared to the control in that specific day (Friedman’s two-way ANOVA by ranks, p < 0.05). Comparisons between test groups (Friedman’s two-way ANOVA by ranks post hoc test, p < 0.05) are available at Supplementary Table 6. Clear symptoms of disturbance were usually observed when subcolonies scored 2 in the score scale. This value was delimited as the Disturbance Threshold (DT), corresponding to the threshold amount of disturbance to become symptomatic.
Insecticide-Treated Fungus-Gardens Are Susceptible to Infection With Escovopsis Conidia
Sulfluramid treatment increased ant mortality from the first day, and after 7 days the majority of ant workers were dying or dead (Figure 4 and Supplementary Table 8). All insecticide-treated subcolonies died by the end of the experiment (Figures 4A,B), meaning that foraging activity was not detected, the fungus garden was decayed, and most ants were dead or dying (Barcoto et al., 2017). For some subcolonies, the fungus garden was scored as decayed at the 7th day, even being overgrowth by Escovopsis, though presenting some survival ants (Figure 4C and Supplementary Table 8). Insecticide-treated subcolonies that were not inoculated with Escovopsis conidia had a significant decrease in healthy conditions and foraging activities already on the first day, dying on day 11 (Figures 4A,B). A similar pattern was observed in insecticide-treated subcolonies inoculated with Escovopsis conidia, which also experienced decline in health conditions on the first day of experiment, scoring as dead on day 7 (Figure 4A). Foraging activities were significantly reduced from the second day and completely interrupted from the third day (Figure 4B). Fungus gardens of these subcolonies were overgrown by the inoculated Escovopsis strains after the subcolony death (Figure 5), presenting also mycelia from non-inoculated Escovopsis strains, Escovopsioides, Syncephalastrum, Fusarium, and Trichoderma (Supplementary Table 6). Even though these subcolonies had died 4 days before the ones treated only with sulfluramid, there were no statistical differences in daily comparisons between them (Friedman’s two-way ANOVA by ranks post hoc test, p > 0.05; Supplementary Table 7).
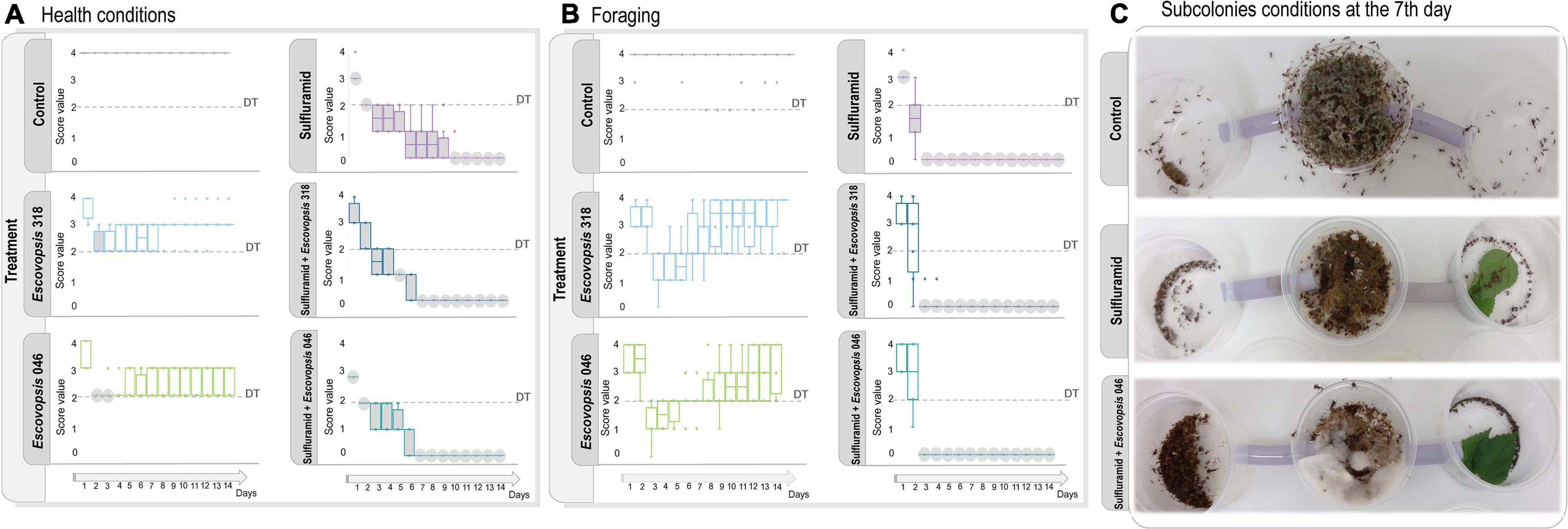
Figure 4. Escovopsis conidia effects in healthy and insecticide-treated Atta sexdens subcolonies. (A) Health conditions and (B) foraging activities after inoculation with Escovopsis strains LESF 046 and LESF 318, scored as defined in Figure 3. Boxes and markers in gray indicate significant differences when compared to the control in that specific day (Friedman’s two-way ANOVA by ranks, p < 0.05). Comparisons between test groups (Friedman’s two-way ANOVA by ranks post hoc test, p < 0.05) are available at Supplementary Table 8. Disturbance Threshold (DT) corresponds to the threshold amount of disturbance for the subcolonies to exhibit disease symptoms. (C) Subcolonies conditions at the 7th day after infection, when most of insecticide-treated subcolonies inoculated with Escovopsis conidia scoring as dead.
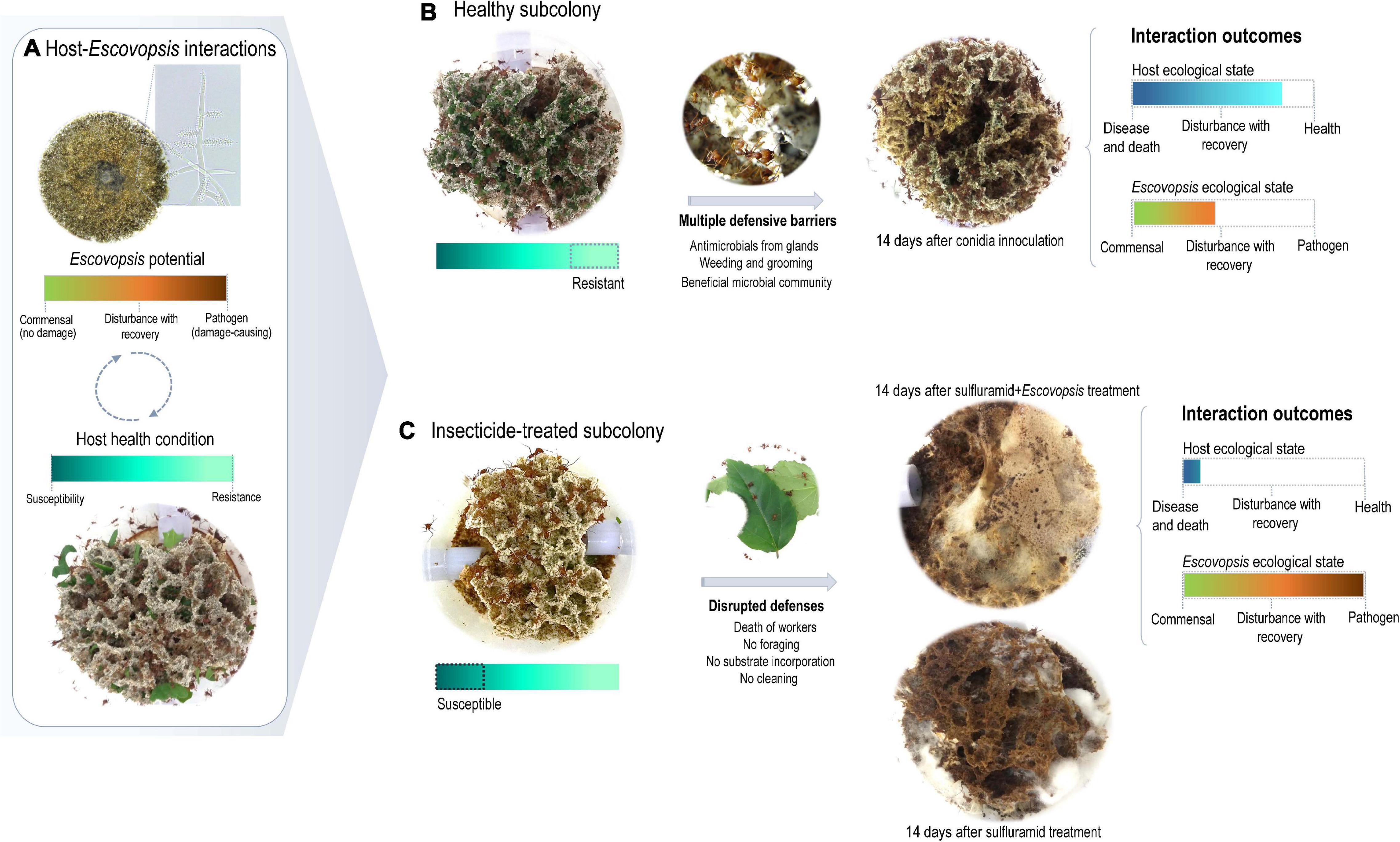
Figure 5. Escovopsis context-dependent pathogenesis. (A) Host-Escovopsis interactions would be a complex outcome deriving from interacting genotypes, phenotypes, and environmental features, resulting in a dynamic continuum ranging from benefits to damage, which would ultimately be modulated by the host susceptibility. (B) A healthy garden includes multiple defenses limiting the infectivity potential of Escovopsis. After 14 days of interaction, the garden shows no signals of infection or disease, being visibly similar to a healthy garden. In such interactions, Escovopsis could be acting as a commensal causing imperceptible damage to the host. (C) Insecticide-treated gardens present several disrupted defenses and the Escovopsis infection is not limited. In this context, Escovopsis would act as a pathogen damaging fungus garden health conditions fast and progressively.
In contrast, none of the healthy subcolonies inoculated with Escovopsis conidia died throughout 14 days of experiment. Although these subcolonies experienced a decline in health conditions after the second day, they gradually recovered and stabilized (Figure 4A). Similarly, foraging activities decreased after the second day of receiving Escovopsis conidia, and were gradually recovered about the seventh (Escovopsis sp. LESF 318 – Acromyrmex sp.) and eighth (Escovopsis sp. LESF 046 – Trachymyrmex sp.) day (Figure 4B).
Discussion
The agricultural system of fungus-growing ants sustains diverse and complex microbial interactions, which determine the ecological success of this insect-microbial symbiosis (Currie et al., 1999a; Mueller et al., 2005; Fernández-Marín et al., 2006; Gerardo et al., 2006b; Poulsen et al., 2010; Suen et al., 2010; Aylward et al., 2012; Boya et al., 2017). As part of a dynamic continuum, whether these interactions would be beneficial, neutral, or harmful depend on the host health and the ecological conditions (Méthot and Alizon, 2014; Figure 5). Escovopsis fungi are reported as parasites of attine ants symbiosis (Currie et al., 1999a; Currie, 2001), and here we explore to what extent the susceptibility of the ants’ fungal cultivars and fungus gardens influence host-Escovopsis interactions. For being more susceptible than L. gongylophorus AS, Leucoagaricus sp. MT tend to be more inhibited by Escovopsis. These outcomes are not related to Escovopsis strains’ origin and interaction patterns, further evidencing that the cultivar susceptibility substantially impacts the interaction results (Figures 1, 2). The susceptibility of the colony (comprising the fungus gardens and ant workers) also seems fundamental in driving interactions outcomes, even determining the recovery and survival of subcolonies. While healthy subcolonies gradually recover from infection with different concentrations of Escovopsis conidia (Figure 3), insecticide-treated subcolonies become susceptible to infection and die within 7 days (Figure 4). However, it is unclear to what extent Escovopsis contributed to the death of subcolonies, which were already damaged by the insecticide effect. Thus, host susceptibility seems to modulate microbial interactions in higher attine symbioses, ultimately determining to what extent the interaction with Escovopsis would render detrimental for the colony (Figure 5).
It is uncertain which biological features make Leucoagaricus sp. MT more susceptible to Escovopsis. We hypothesize that susceptibility differences between L. gongylophorus AS and Leucoagaricus sp. MT could reflect the effectiveness of defensive mechanisms. Such approaches could involve fungal symbiont-secreted chitinases to avoid invasive microbes (Erthal et al., 2009), and laccases (Aylward et al., 2013; De Fine Licht et al., 2013) to detoxify Escovopsis metabolites with antimicrobial properties (Dean and Eriksson, 1994; Folgarait et al., 2011; Becker et al., 2016; Divya and Sadasivan, 2016; Boya et al., 2017; Dhodary et al., 2018; Heine et al., 2018). It is possible that the low laccase activity (De Fine Licht et al., 2013) would render Leucoagaricus sp. MT more susceptible to Escovopsis metabolites. Fungal cultivar laccases could also be involved in biosynthesis of heterogeneous melanin, known for protecting hyphae from toxins and hydrolytic enzymes (as chitinases and glucanases). Melanin accumulation results in a characteristic dark pigmentation with antimicrobial properties at interaction zones, which could be occurring in in vitro assays (Bull, 1970; Bell and Wheeler, 1986; Boddy and Hiscox, 2017; Figures 1C,D). Another potential defensive mechanism includes the biosynthesis of antimicrobial metabolites by the fungal cultivar (Hervey and Nair, 1979; Wang et al., 1999), which would partially explain the Escovopsis inhibition observed in 21% of in vitro interactions (Figure 1). However, these putative mechanisms seem not enough to outcompete Escovopsis growth, which was attracted to both fungal cultivars and inhibited their growth in the majority of FEI (Figure 1). It is worthy to point that fungal cultivar inhibition is not an outcome particular to interactions with Escovopsis, as it also results from in vitro interactions between cultivar and other filamentous fungi (Ortiz and Orduz, 2001; Silva et al., 2006; Barcoto et al., 2017; do Nascimento et al., 2017; Bizarria et al., 2018). Instead of being considered as a consequence of Escovopsis pathogenicity (Currie, 2001; Currie et al., 2003; Reynolds and Currie, 2004; Folgarait et al., 2011; Varanda-Haifig et al., 2017), we suggest that such outcomes also integrate the fungal cultivar responses. These fungal-fungal interactions would depend on to what extent the fungal cultivar and Escovopsis respond to and are affected by each other. Therefore, Leucoagaricus sp. MT would be less effective in inhibiting Escovopsis and more affected throughout the interaction.
Fungus gardens susceptibility may include several other players and factors influencing FEI dynamics. Thus, outcomes observed in vitro are not fully replicated, as only 5% of the subcolonies died when infected with Escovopsis. Although Escovopsis treatment damaged the garden at some extent, the majority of healthy subcolonies were able to gradually recover within 14 days (Figure 3). Such damage may result from both direct effects of Escovopsis growth and the workers’ effort to eliminate the high concentration of inoculated conidia, since fungus garden grooming and weeding increase when colonies face fungal infections (Currie, 2001; Currie and Stuart, 2001; Barcoto et al., 2017; Nilsson-Møller et al., 2018). As weeding implies the removal of fungus garden fragments, this defensive strategy could also reduce the garden lifetime. The initial damage followed by recovery could reflect multiple defensive barriers presented by a healthy colony, imposing limitations for the infectivity potential of Escovopsis (Figure 5). Defensive barriers to avoid accumulating damage would include: (i) ant workers cleaning and sanitizing behaviors (Currie and Stuart, 2001; Fernández-Marín et al., 2006); (ii) potential detoxification and antimicrobial mechanisms by the fungal cultivar (Hervey and Nair, 1979; Bell and Wheeler, 1986; Wang et al., 1999; Divya and Sadasivan, 2016; Boddy and Hiscox, 2017); and (iii) the garden microbiota (Santos et al., 2004; Egan and Gardiner, 2016; Longford et al., 2019; Barcoto et al., 2020).
When some of these defensive barriers are disrupted, for instance by insecticide treatment, the healthy functioning of the entire system appears to collapse (Figures 4, 5). Sulfluramid (N-ethyl perfluorooctane sulfonamide) is widely employed for eliminating leaf-cutting ant species in the field in Brazil. This insecticide acts by interrupting electron flow through the mitochondrial matrix, ultimately preventing aerobic respiration and causing the ants’ death (Boaretto and Forti, 1997; Della Lucia et al., 2014). With an increasing mortality of ants, multiple chemical and behavioral defenses would be simultaneously put down. For Atta ants, reducing the number of tending workers imply a decrease in glandular antimicrobial compounds (Fernández-Marín et al., 2006), as well as a lower frequency of fungus grooming and weeding for controlling and removing harmful microorganisms (Currie and Stuart, 2001; Barcoto et al., 2017; Nilsson-Møller et al., 2018). Atta species apparently lost cuticular Actinobacteria symbionts over the evolutionary time (Currie et al., 2006; Li et al., 2018), possibly relying on alternative behavioral and chemical defenses to control the growth of infectious microbes (Currie and Stuart, 2001; Fernández-Marín et al., 2009; Fernández-Marín et al., 2013; Yek et al., 2012). As a direct consequence of ant mortality, the absence of cleaning strategies would allow the spread of pathogens throughout the garden. In contrast, for the majority of other attine genera that present cuticular Actinobacteria, these symbionts may aid to the complexity of host-pathogen dynamics in the fungus garden (Sen et al., 2009; Goldstein and Klassen, 2020). These filamentous bacteria, mainly in the genera Pseudonocardia and Amycolatopsis, produce broad spectrum antimicrobial compounds thought to defend the workers and the fungus garden against diverse fungal antagonists, including Escovopsis (Currie et al., 1999b; Oh et al., 2009; Sen et al., 2009; Dângelo et al., 2016; Goldstein and Klassen, 2020). It would be very informative to evaluate host-Escovopsis dynamics throughout the attine group, comparing outcomes of interactions on Actinobacteria-hosting and non-hosting ant genera.
Ants mortality is accompanied by decrease in foraging activities (Figure 4), implying substantial reduction in plant substrate incorporation, which could affect the fungal cultivar metabolic activity (Schiøtt et al., 2010; Moller et al., 2011). Feeding a “vicious circle” (Beldomenico and Begon, 2010), this would impact the ants’ nutrition (Silva et al., 2003) and environmental conditions important for microbial community assembling (Barcoto et al., 2020). When reaching a threshold amount of disturbance, the fungus garden would be susceptible to virulence traits (i.e., features causing disease), exhibiting disease symptoms (Casadevall and Pirofski, 2001, 2003). Virulence traits would emerge from SEI only when the host health was previously disturbed (Casadevall and Pirofski, 2001, 2015; Brown et al., 2012; Méthot and Alizon, 2014). Under these circumstances, Escovopsis secondary compounds (Boya et al., 2017; Dhodary et al., 2018) and enzymes for metabolizing the mycelia of fungal cultivars (de Man et al., 2016), would enhance its infectivity and induce disease. Since the disease progressed only when the subcolony health was already impaired, Escovopsis infections seem to have an opportunistic nature (Casadevall and Pirofski, 1999; Brown et al., 2012; Méthot and Alizon, 2014; Casadevall and Pirofski, 2015). Rather than the effect of a single microbial agent, diseases in higher attine fungiculture could be a consequence of disturbances in the healthy microbial community (including the fungal cultivar; Casadevall and Pirofski, 2015; Egan and Gardiner, 2016; Biedermann and Rohlfs, 2017; Bass et al., 2019). Environmental disturbances would increase the fungus garden susceptibility, allowing the transition of commensals, saprotrophs, and opportunistic microbes to a pathogen mode (Méthot and Alizon, 2014; Egan and Gardiner, 2016; Bass et al., 2019). Therefore, when previously treated with sulfluramid, subcolonies were highly susceptible to Escovopsis infections, with health conditions impairing fast and progressively to death (Figures 4, 5). It is unclear to what extent and by which mechanisms Escovopsis negatively affected already debilitated subcolonies (Supplementary Table 7). Plausibly, other fungi reported in fungus gardens (as non-inoculated Escovopsis, Escovopsioides, Syncephalastrum, Fusarium, and Trichoderma, Supplementary Table 6) could have a similar effect in such conditions. Also, these fungi could add to the potential negative impact of Escovopsis, contributing to a cumulative detrimental situation.
Escovopsis has the capacity to metabolize diverse carbon sources (de Man et al., 2016). This would allow Escovopsis to function as a context-dependent commensal in healthy fungus gardens, potentially exploiting carbohydrates derived from the fungal cultivar metabolism (Gomes De Siqueira et al., 1998; Silva et al., 2003, Silva et al., 2006; Moller et al., 2011; Mathis and Bronstein, 2020). Escovopsis growth and infectivity would be limited by Atta ants’ cleaning and sanitizing behavior, as well as by the cultivar and the microbial community defenses. Environmental stresses or fungus-garden senescence would weaken the colonies defensive mechanisms, altering the interactions within the microbial community and ultimately enhancing host susceptibility (Casadevall and Pirofski, 2015; Egan and Gardiner, 2016; Casadevall, 2017; Bass et al., 2019). In such circumstances, Escovopsis growth would not be limited either by the ants, the fungal cultivar, or the microbial community. When the host becomes susceptible, the Escovopsis pathogenic transition would be triggered (Pitlik and Koren, 2017; Bass et al., 2019), enhancing its infectivity. Escovopsis pathogenicity would be based on degradation of the fungal cultivar mycelia (Reynolds and Currie, 2004) and production of metabolites toxic to the workers and the cultivar (de Man et al., 2016; Boya et al., 2017; Dhodary et al., 2018; Heine et al., 2018). For Atta fungus gardens, these effects could be correlated to decreasing in fungus garden biomass, amount of workers and brood reported as consequence of Escovopsis infections (Currie, 2001). Alternatively, as Escovopsis codifies for amylolytic and β-glucanolytic hydrolases, these enzymes would help in outcompeting the fungal symbiont for starch and glucans as carbon sources (Silva et al., 2006; Erthal et al., 2009; De Fine Licht and Boomsma, 2010; Moller et al., 2011), further inhibiting the fungal cultivar growth. In addition, older parts of the garden appear to stimulate Escovopsis conidia germination (Augustin et al., 2017), raising the possibility of conidia remaining dormant while the fungus garden is healthy, then germinating as the garden becomes older.
It remains to be evaluated the putative role of Escovopsis as a context-dependent commensal or saprotroph, as well as the metabolic processes behind the eventual pathogenic transition (Chamberlain et al., 2014; Bass et al., 2019; Mathis and Bronstein, 2020). Whether and which physiological processes could simultaneously sustain Escovopsis as a commensal and participate in the disease process (for instance, the chitinolytic and amylolytic capacity) are open research windows. It also remains to be investigated the resilience of A. sexdens symbiosis when interacting with Escovopsis, to define how much damage the colony tolerates before rendering a visible infection (Casadevall and Pirofski, 2015). Its worthy to consider that marked differences in caste and division of labor (Wilson, 1980), chemical and behavioral defensive strategies (Currie and Stuart, 2001; Fernández-Marín et al., 2006), and the apparent absence of cuticular Actinobacteria symbionts (Currie et al., 2006; Li et al., 2018) may render particular host-pathogen interactions in the Atta symbiosis. Whether and how patterns of host-modulated pathogenesis would emerge from complex interactions involving ant-Actinobacteria-fungal cultivar-Escovopsis in Actinobacteria-hosting attines is yet to be verified. A growing understanding of infectious diseases as outcomes of complex host-microbial interactions has evidenced that commensals and saprotrophs have the potential to turn into pathogens when having the opportunity to do so (Méthot and Alizon, 2014; Casadevall, 2017; Pitlik and Koren, 2017; Bass et al., 2019). For A. sexdens colonies-Escovopsis interactions, our findings suggest that the opportunity to become a disease-causing agent is modulated largely by the susceptibility of the fungal cultivar, the ants and the fungus garden.
Data Availability Statement
The datasets presented in this study can be found in online repositories. The names of the repository/repositories and accession number(s) can be found in the article/Supplementary Material.
Author Contributions
IJG designed the experimental setup, carried out the assays, the data analysis, drafted initial versions of the manuscript text and figures discussed the results, and contributed to the manuscript writting. MB designed the experimental setup, conceived the figures, discussed the results, and wrote the manuscript. QM carried out the assays and data analysis, discussed the results, and contributed to the manuscript writing. AG carried out the assays, discussed the results, and contributed to the manuscript writing. LM carried out the assays and data analysis. OB provided the ant colonies for the experiments, provided expertise in rearing ant colonies in the lab, discussed the results, and contributed to the manuscript writing. AR designed the experimental setup, assisted with results interpretation and discussion, and wrote the manuscript. All authors contributed to the article and approved the submitted version.
Funding
The authors would like to thank “FAPESP – Fundação de Amparo à Pesquisa do Estado de São Paulo” for providing financial support (grants #2017/12689-4 and #2019/03746-0). IJG was a recipient of a scholarship from “CNPq – Conselho Nacional de Desenvolvimento Científico e Tecnológico” under the CNPq/PEC-PG program (scholarship #190502/2014-2). AR would like to thank CNPq for providing financial support (grants #305341/2015-4 and #305269/2018-6).
Conflict of Interest
The authors declare that the research was conducted in the absence of any commercial or financial relationships that could be construed as a potential conflict of interest.
Acknowledgments
The authors would like to thank Fernando Carlos Pagnocca for providing the fungal cultivar isolate FF2006; Julio Osti for technical assistance; Alvaro Neto and Maryana Nogueira for assistance with the in vivo experiments; Tássio Brito de Oliveira and Dennis Denis Ávila for helping with statistical analysis; Silma Rocha for valuable suggestions on the experimental design, and Pepijn Kooij for valuable comments on the manuscript.
Supplementary Material
The Supplementary Material for this article can be found online at: https://www.frontiersin.org/articles/10.3389/fmicb.2021.673444/full#supplementary-material
References
Antonovics, J., Boots, M., Ebert, D., Koskella, B., Poss, M., and Sadd, B. M. (2013). The origin of specificity by means of natural selection: evolved and nonhost resistance in host–pathogen interactions. Evolution 67, 1–9. doi: 10.1111/j.1558-5646.2012.01793.x
Augustin, J. O., Simões, T. G., Dijksterhuis, J., Elliot, S. L., and Evans, H. C. (2017). Putting the waste out: a proposed mechanism for transmission of the mycoparasite Escovopsis between leafcutter ant colonies. Roy. Soc. Open Sci. 4:161013. doi: 10.1098/rsos.161013
Aylward, F. O., Burnum, K. E., Scott, J. J., Suen, G., Tringe, S. G., Adams, S. M., et al. (2012). Metagenomic and metaproteomic insights into bacterial communities in leaf-cutter ant fungus gardens. ISME J. 6, 1688–1701.
Aylward, F. O., Burnum-Johnson, K. E., Tringe, S. G., Teiling, C., Tremmel, D. M., Moeller, J. A., et al. (2013). Leucoagaricus gongylophorus produces diverse enzymes for the degradation of recalcitrant plant polymers in leaf-cutter ant fungus gardens. Appl. Env. Microbiol. 79, 3770–3778. doi: 10.1128/AEM.03833-12
Barcoto, M. O., Carlos-Shanley, C., Fan, H., Ferro, M., Nagamoto, N. S., Bacci, M., et al. (2020). Fungus-growing insects host a distinctive microbiota apparently adapted to the fungiculture environment. Sci. Rep. 10:12384. doi: 10.1038/s41598-020-68448-68447
Barcoto, M. O., Pedrosa, F., Bueno, O. C., and Rodrigues, A. (2017). Pathogenic nature of Syncephalastrum in Atta sexdens rubropilosa fungus gardens. Pest Manag. Sci. 73, 999–1009. doi: 10.1002/ps.4416
Bass, D., Stentiford, G. D., Wang, H.-C., Koskella, B., and Tyler, C. R. (2019). The pathobiome in animal and plant diseases. Trends Ecol. Evol. 34, 996–1008.
Becker, D., Della Giustina, S. V., Rodriguez-Mozaz, S., Schoevaart, R., Barceló, D., de Cazes, M., et al. (2016). Removal of antibiotics in wastewater by enzymatic treatment with fungal laccase–degradation of compounds does not always eliminate toxicity. Bio. Tech. 219, 500–509. doi: 10.1016/j.biortech.2016.08.004
Beldomenico, P. M., and Begon, M. (2010). Disease spread, susceptibility and infection intensity: vicious circles? Trends Ecol. Evol. 25, 21–27.
Bell, A. A., and Wheeler, M. H. (1986). Biosynthesis and functions of fungal melanins. Ann. Rev. Phytopathol. 24, 411–451.
Best, A., White, A., and Boots, M. (2009). The implications of coevolutionary dynamics to host-parasite interactions. Am. Nat. 173, 779–791.
Biedermann, P. H., and Rohlfs, M. (2017). Evolutionary feedbacks between insect sociality and microbial management. Curr. Op. Ins. Sci. 22, 92–100. doi: 10.1016/j.cois.2017.06.003
Birnbaum, S. S. L., and Gerardo, N. M. (2016). Patterns of specificity of the pathogen Escovopsis across the fungus-growing ant symbiosis. Am. Nat. 188, 52–65.
Bizarria, R., Moia, I. C., Montoya, Q. V., Polezel, D. A., and Rodrigues, A. (2018). Soluble compounds of filamentous fungi harm the symbiotic fungus of leafcutter ants. Curr. Microbiol. 75, 1602–1608. doi: 10.1007/s00284-018-1566-1
Boaretto, M. A. C., and Forti, L. C. (1997). Perspectivas no controle de formigas cortadeiras. Sér Téc IPEF 11, 31–46.
Boddy, L., and Hiscox, J. (2017). “Fungal ecology: principles and mechanisms of colonization and competition by saprotrophic fungi,” in The Fungal Kingdom, eds J. Hetiman, B. J. Howlett, P. W. Crous, E. H. Stukenbrock, T. Y. James, and N. A. R. Gow (Washington, DC: ASM Press), 293–308. doi: 10.1128/9781555819583.ch13
Boya, C. P., Fernández-Marín, H., Mejía, L. C., Spadafora, C., Dorrestein, P. C., and Gutiérrez, M. (2017). Imaging mass spectrometry and MS/MS molecular networking reveals chemical interactions among cuticular bacteria and pathogenic fungi associated with fungus-growing ants. Sci. Rep. 7, 5604–5604. doi: 10.1038/s41598-017-05515-6
Bratburd, J. R., Arango, R. A., and Horn, H. A. (2020). Defensive symbioses in social insects can inform human health and agriculture. Front. Microbiol. 11:76. doi: 10.3389/fmicb.2020.00076
Brown, S. P., Cornforth, D. M., and Mideo, N. (2012). Evolution of virulence in opportunistic pathogens: generalism, plasticity, and control. Trends Micro. 20, 336–342. doi: 10.1016/j.tim.2012.04.005
Bull, A. T. (1970). Inhibition of polysaccharases by melanin: enzyme inhibition in relation to mycolysis. Arch. Bioche. Biophy. 137, 345–356.
Carreiro, S. C., Pagnocca, F. C., Bueno, O. C., Júnior, M. B., Hebling, M. J. A., and da Silva, O. A. (1997). Yeasts associated with nests of the leaf-cutting ant Atta sexdens rubropilosa Forel, 1908. Antonie Van Leeuwenhoek 71, 243–248. doi: 10.1023/A:1000182108648
Casadevall, A. (2017). The pathogenic potential of a microbe. mSphere 2:e00015-17. doi: 10.1128/mSphere.00015-17
Casadevall, A., and Pirofski, L. (1999). Host-Pathogen interactions: redefining the basic concepts of virulence and pathogenicity. Infect. Immun. 67:3703–3713. doi: 10.1128/IAI.67.8.3703-3713.1999
Casadevall, A., and Pirofski, L. (2001). Host-Pathogen interactions: the attributes of virulence. J. Infect. Dis. 184, 337–344. doi: 10.1086/322044
Casadevall, A., and Pirofski, L. (2003). The damage-response framework of microbial pathogenesis. Nat. Rev. Microbiol. 1, 17–24. doi: 10.1038/nrmicro732
Casadevall, A., and Pirofski, L. (2015). What is a host? incorporating the microbiota into the damage-response framework. Infect. Immun. 83:2.
Casadevall, A., and Pirofski, L. (2018). What is a host? attributes of individual susceptibility. Infect. Immun. 86:e00636-17.
Chamberlain, S. A., Bronstein, J. L., and Rudgers, J. A. (2014). How context dependent are species interactions? Ecol. Lett. 17, 881–890. doi: 10.1111/ele.12279
Currie, C. R. (2001). Prevalence and impact of a virulent parasite on a tripartite mutualism. Oecologia 128, 99–106. doi: 10.1007/s004420100630
Currie, C. R., Mueller, U. G., and Malloch, D. (1999a). The agricultural pathology of ant fungus gardens. Proc. Natl. Acad. Sci. U S A. 96:7998. doi: 10.1073/pnas.96.14.7998
Currie, C. R., Scott, J. A., Summerbell, R. C., and Malloch, D. (1999b). Fungus-growing ants use antibiotic-producing bacteria to control garden parasites. Nature 398, 701–704.
Currie, C. R., and Stuart, A. E. (2001). Weeding and grooming of pathogens in agriculture by ants. Proc. R. Soc. B. 268, 1033–1039. doi: 10.1098/rspb.2001.1605
Currie, C. R., Wong, B., Stuart, A. E., Schultz, T. R., Rehner, S. A., Mueller, U. G., et al. (2003). Ancient tripartite coevolution in the attine ant-microbe symbiosis. Science 299:386. doi: 10.1126/science.1078155
Currie, C. R., Poulsen, M., Mendenhall, J., Boomsma, J. J., and Billen, J. (2006). Coevolved crypts and exocrine glands support mutualistic bacteria in fungus-growing ants. Science 311, 81–83. doi: 10.1126/science.1119744
Dângelo, R. A. C., de Souza, D. J., Mendes, T. D., Couceiro, J. D. C., and Lucia, T. M. C. D. (2016). Actinomycetes inhibit filamentous fungi from the cuticle of Acromyrmex leafcutter ants. J. Basic Microbiol. 56, 229–237.
Darriba, D., Taboada, G. L., Doallo, R., and Posada, D. (2012). jModelTest 2: more models, new heuristics and parallel computing. Nat. Meth. 9, 772–772.
De Fine Licht, H. H., and Boomsma, J. J. (2010). Forage collection, substrate preparation, and diet composition in fungus-growing ants. Ecol. Entomol. 35, 259–269. doi: 10.1111/j.1365-2311.2010.01193.x
De Fine Licht, H. H., Boomsma, J. J., and Tunlid, A. (2014). Symbiotic adaptations in the fungal cultivar of leaf-cutting ants. Nat. Commun. 5:5675. doi: 10.1038/ncomms6675
De Fine Licht, H. H., Schiøtt, M., Rogowska-Wrzesinska, A., Nygaard, S., Roepstorff, P., and Boomsma, J. J. (2013). Laccase detoxification mediates the nutritional alliance between leaf-cutting ants and fungus-garden symbionts. Proc. Natl. Acad. Sci. U S A. 110, 583–587. doi: 10.1073/pnas.1212709110
de Man, T. J. B., Stajich, J. E., Kubicek, C. P., Teiling, C., Chenthamara, K., Atanasova, L., et al. (2016). Small genome of the fungus Escovopsis weberi, a specialized disease agent of ant agriculture. Proc. Natl. Acad. Sci. U S A. 113:3567. doi: 10.1073/pnas.1518501113
Dean, J. F., and Eriksson, K.-E. L. (1994). Laccase and the deposition of lignin in vascular plants. Holzforschung 48:21.
Della Lucia, T. M. C., Gandra, L. C., and Guedes, R. N. M. C. (2014). Managing leaf-cutting ants: peculiarities, trends and challenges. Pest Manag. Sci. 70, 14–23. doi: 10.1002/ps.3660
Dhodary, B., Schilg, M., Wirth, R., and Spiteller, D. (2018). Secondary metabolites from Escovopsis weberi and their role in attacking the garden fungus of leaf-cutting ants. Chem. Euro. J. 24, 4445–4452. doi: 10.1002/chem.201706071
Divya, L., and Sadasivan, C. (2016). Trichoderma viride laccase plays a crucial role in defense mechanism against antagonistic organisms. Front. Microbiol. 7:741. doi: 10.3389/fmicb.2016.00741
do Nascimento, M. O., de Almeida Sarmento, R., dos Santos, G. R., de Oliveira, C. A., and de Souza, D. J. (2017). Antagonism of Trichoderma isolates against Leucoagaricus gongylophorus (Singer) Möller. J. Basic Microbiol. 57, 699–704. doi: 10.1002/jobm.201600755
Egan, S., and Gardiner, M. (2016). Microbial dysbiosis: rethinking disease in marine ecosystems. Front. Microbiol. 7:991. doi: 10.3389/fmicb.2016.00991
Erthal, M. Jr., Silva, C. P., Cooper, R. M., and Samuels, R. I. (2009). Hydrolytic enzymes of leaf-cutting ant fungi. Comp. Bioche. Phys. B 152, 54–59.
Fernández-Marín, H., Bruner, G., Gomez, E. B., Nash, D. R., Boomsma, J. J., and Wcislo, W. T. (2013). Dynamic disease management in Trachymyrmex fungus-growing ants (Attini: Formicidae). Am. Nat. 181, 571–582. doi: 10.1086/669664
Fernández-Marín, H., Zimmerman, J. K., Nash, D. R., Boomsma, J. J., and Wcislo, W. T. (2009). Reduced biological control and enhanced chemical pest management in the evolution of fungus farming in ants. Proc. R. Soc. B 276, 2263–2269. doi: 10.1098/rspb.2009.0184
Fernández-Marín, H., Zimmerman, J. K., Rehner, S. A., and Wcislo, W. T. (2006). Active use of the metapleural glands by ants in controlling fungal infection. Proc. R. Soc. B 273, 1689–1695. doi: 10.1098/rspb.2006.3492
Fisher, P. J., Stradling, D. J., Sutton, B. C., and Petrini, L. E. (1996). Microfungi in the fungus gardens of the leaf-cutting ant Atta cephalotes: a preliminary study. Myco. Res. 100, 541–546. doi: 10.1016/S0953-7562(96)80006-80002
Folgarait, P. J., Marfetán, J. A., and Cafaro, M. J. (2011). Growth and conidiation response of Escovopsis weberi (Ascomycota: Hypocreales) against the fungal cultivar of Acromyrmex lundii (Hymenoptera: Formicidae). Envir. Entomol. 40, 342–349. doi: 10.1603/EN10111
Frey-Klett, P., Burlinson, P., Deveau, A., Barret, M., Tarkka, M., and Sarniguet, A. (2011). Bacterial-Fungal interactions: hyphens between agricultural, clinical, environmental, and food microbiologists. Microbiol. Mol. Biol. Rev. 75:583. doi: 10.1128/MMBR.00020-11
Gerardo, N. M., Jacobs, S. R., Currie, C. R., and Mueller, U. G. (2006a). Ancient host–pathogen associations maintained by specificity of chemotaxis and antibiosis. PLoS Biol. 4:e235. doi: 10.1371/journal.pbio.0040235
Gerardo, N. M., Mueller, U. G., and Currie, C. R. (2006b). Complex host-pathogen coevolution in the Apterostigma fungus-growing ant-microbe symbiosis. BMC Evol. Biol. 6:88. doi: 10.1186/1471-2148-6-88
Gerardo, N. M., Mueller, U. G., Price, S. L., and Currie, C. R. (2004). Exploiting a mutualism: parasite specialization on cultivars within the fungus–growing ant symbiosis. Proc. R. Soc. B 271, 1791–1798. doi: 10.1098/rspb.2004.2792
Gomes De Siqueira, C., Bacci, M., Pagnocca, F. C., Bueno, O. C., and Hebling, M. J. (1998). Metabolism of plant polysaccharides by Leucoagaricus gongylophorus, the symbiotic fungus of the leaf-cutting ant Atta sexdens L. Appl. Env. Microbiol. 64:4820. doi: 10.1128/AEM.64.12.4820-4822.1998
Gonze, D., Coyte, K. Z., Lahti, L., and Faust, K. (2018). Microbial communities as dynamical systems. Curr. Opin. Microbiol. 44, 41–49. doi: 10.1016/j.mib.2018.07.004
Goldstein, S. L., and Klassen, J. L. (2020). Pseudonocardia symbionts of fungus-growing ants and the evolution of defensive secondary metabolism. Front. Microbiol. 11:621041. doi: 10.3389/fmicb.2020.621041
Hammer, Ø, Harper, D. A., and Ryan, P. D. (2001). PAST: paleontological statistics software package for education and data analysis. Palaeontol. Electron. 4:9.
Heine, D., Holmes, N. A., Worsley, S. F., Santos, A. C. A., Innocent, T. M., Scherlach, K., et al. (2018). Chemical warfare between leafcutter ant symbionts and a co-evolved pathogen. Nat. Commun. 9:2208. doi: 10.1038/s41467-018-04520-1
Hervey, A., and Nair, M. S. R. (1979). Antibiotic metabolite of a fungus cultivated by gardening ants. Mycologia 71, 1064–1066.
Katoh, K., and Standley, D. M. (2013). MAFFT multiple sequence alignment software version 7: improvements in performance and usability. Mol. Bio. Evol. 30, 772–780. doi: 10.1093/molbev/mst010
Lambrechts, L., Fellous, S., and Koella, J. C. (2006). Coevolutionary interactions between host and parasite genotypes. Trends Parasitol. 22, 12–16. doi: 10.1016/j.pt.2005.11.008
Li, H., Sosa-Calvo, J., Horn, H. A., Pupo, M. T., Clardy, J., Rabeling, C., et al. (2018). Convergent evolution of complex structures for ant–bacterial defensive symbiosis in fungus-farming ants. Proc. Natl. Acad. Sci. U S A. 115, 10720–10725. doi: 10.1073/pnas.1809332115
Little, T. J., Shuker, D. M., Colegrave, N., Day, T., and Graham, A. L. (2010). The coevolution of virulence: tolerance in perspective. PLoS Pathol. 6:e1001006. doi: 10.1371/journal.ppat.1001006
Longford, S. R., Campbell, A. H., Nielsen, S., Case, R. J., Kjelleberg, S., and Steinberg, P. D. (2019). Interactions within the microbiome alter microbial interactions with host chemical defences and affect disease in a marine holobiont. Sci. Rep. 9:1363. doi: 10.1038/s41598-018-37062-z
Marfetán, J. A., Romero, A. I., and Folgarait, P. J. (2015). Pathogenic interaction between Escovopsis weberi and Leucoagaricus sp.: mechanisms involved and virulence levels. Fun. Ecol. 17, 52–61. doi: 10.1016/j.funeco.2015.04.002
Martin, M. M. (1970). The biochemical basis of the fungus-attine ant symbiosis. Science 169:16. doi: 10.1126/science.169.3940.16
Mathis, K. A., and Bronstein, J. L. (2020). Our current understanding of commensalism. Annu. Rev. Ecol. Evol. Syst. 51, 167–189. doi: 10.1146/annurev-ecolsys-011720-040844
Meirelles, L. A., Solomon, S. E., Bacci, M., Wright, A. M., Mueller, U. G., and Rodrigues, A. (2015). Shared Escovopsis parasites between leaf-cutting and non-leaf-cutting ants in the higher attine fungus-growing ant symbiosis. Roy. Soc. Op. Sci. 2:150257. doi: 10.1098/rsos.150257
Méthot, P.-O., and Alizon, S. (2014). What is a pathogen? toward a process view of host-parasite interactions. Virulence 5, 775–785. doi: 10.4161/21505594.2014.960726
Moller, I. E., De Fine Licht, H. H., Harholt, J., Willats, W. G. T., and Boomsma, J. J. (2011). The dynamics of plant cell-wall polysaccharide decomposition in leaf-cutting ant fungus gardens. PLoS One 6:e17506. doi: 10.1371/journal.pone.0017506
Montoya, Q. V., Martiarena, M. J. S., Polezel, D. A., Kakazu, S., and Rodrigues, A. (2019). More pieces to a huge puzzle: two new Escovopsis species from fungus gardens of attine ants. MycoKeys 46, 97–118. doi: 10.3897/mycokeys.46.30951
Mueller, U. G., Gerardo, N. M., Aanen, D. K., Six, D. L., and Schultz, T. R. (2005). The evolution of agriculture in insects. Ann. Rev. Ecol. Evol. Syst. 36, 563–595. doi: 10.1146/annurev.ecolsys.36.102003.152626
Mueller, U. G., Ishak, H. D., Bruschi, S. M., Smith, C. C., Herman, J. J., Solomon, S. E., et al. (2017). Biogeography of mutualistic fungi cultivated by leafcutter ants. Mol. Ecol. 26, 6921–6937. doi: 10.1111/mec.14431
Newmeyer, D. (1990). Filtering small quantities of conidial suspensions to remove mycelial fragments. Fungal Genet. Rep. 37:15.
Nilsson-Møller, S., Poulsen, M., and Innocent, T. M. (2018). A visual guide for studying behavioral defenses to pathogen attacks in leaf-cutting ants. J. Vis. Exp. 140:e58420. doi: 10.3791/58420
Nixon, K. C. (1999). WinClada ver. 1.0000. Available online at: www.cladistics.org (accessed April 14, 2019).
Oh, D.-C., Poulsen, M., Currie, C. R., and Clardy, J. (2009). Dentigerumycin: a bacterial mediator of an ant-fungus symbiosis. Nat. Chem. Biol. 5, 391–393. doi: 10.1038/nchembio.159
Ortiz, A., and Orduz, S. (2001). In vitro evaluation of Trichoderma and Gliocladium antagonism against the symbiotic fungus of the leaf-cutting ant Atta cephalotes. Mycopathologia 150, 53–60.
Osti, J. F., and Rodrigues, A. (2018). Escovopsioides as a fungal antagonist of the fungus cultivated by leafcutter ants. BMC Microbiol. 18, 130. doi: 10.1186/s12866-018-1265-x
Pagnocca, F., Silva, O., Hebling-Beraldo, M., Bueno, O., Fernandes, J., and Vieria, P. (1990). Toxicity of sesame extracts to the leaf cutting ants symbiotic fungus. Bull. Entomol. Res. 80, 349–352.
Pitlik, S. D., and Koren, O. (2017). How holobionts get sick—toward a unifying scheme of disease. Microbiome 5:64. doi: 10.1186/s40168-017-0281-287
Poulsen, M., Cafaro, M. J., Erhardt, D. P., Little, A. E., Gerardo, N. M., Tebbets, B., et al. (2010). Variation in Pseudonocardia antibiotic defence helps govern parasite-induced morbidity in Acromyrmex leaf-cutting ants. Env. Microbiol. Rep. 2, 534–540. doi: 10.1111/j.1758-2229.2009.00098.x
Quinlan, R. J., and Cherrett, J. M. (1979). The role of fungus in the diet of the leaf-cutting ant Atta cephalotes (L.). Ecol. Entomol. 4, 151–160. doi: 10.1111/j.1365-2311.1979.tb00570.x
Restif, O., and Koella, J. C. (2003). Shared control of epidemiological traits in a coevolutionary model of host-parasite interactions. Am. Nat. 161, 827–836. doi: 10.1086/375171
Reynolds, H. T., and Currie, C. R. (2004). Pathogenicity of Escovopsis weberi: the parasite of the attine ant-microbe symbiosis directly consumes the ant-cultivated fungus. Mycologia 96, 955–959. doi: 10.1080/15572536.2005.11832895
Rodrigues, A., Bacci, M. Jr., Mueller, U. G., Ortiz, A., and Pagnocca, F. C. (2008). Microfungal “weeds” in the leafcutter ant symbiosis. Microb. Ecol. 56, 604–614. doi: 10.1007/s00248-008-9380-9380
Ronquist, F., Teslenko, M., Van Der Mark, P., Ayres, D. L., Darling, A., Höhna, S., et al. (2012). MrBayes 3.2: efficient Bayesian phylogenetic inference and model choice across a large model space. Syst. Biol. 61, 539–542.
Santos, A. V., Dillon, R. J., Dillon, V. M., Reynolds, S. E., and Samuels, R. I. (2004). Occurrence of the antibiotic producing bacterium Burkholderia sp. in colonies of the leaf-cutting ant Atta sexdens rubropilosa. FEMS Microbiol. Lett. 239, 319–323. doi: 10.1016/j.femsle.2004.09.005
Schiøtt, M., Rogowska-Wrzesinska, A., Roepstorff, P., and Boomsma, J. J. (2010). Leaf-cutting ant fungi produce cell wall degrading pectinase complexes reminiscent of phytopathogenic fungi. BMC Biol. 8:156. doi: 10.1186/1741-7007-8-156
Schneider, C., Rasband, W., and Eliceiri, K. (2012). NIH Image to ImageJ: 25 years of image analysis. Nat. Methods 9, 671–675. doi: 10.1038/nmeth.2089
Sen, R., Ishak, H. D., Estrada, D., Dowd, S. E., Hong, E., and Mueller, U. G. (2009). Generalized antifungal activity and 454-screening of Pseudonocardia and Amycolatopsis bacteria in nests of fungus-growing ants. Proc. Natl. Acad. Sci. U S A. 106:17805. doi: 10.1073/pnas.0904827106
Silva, A. Jr., Bacci, M., de Siqueira, C. G., Bueno, O. C., Pagnocca, F. C., and Hebling, M. J. A. (2003). Survival of Atta sexdens workers on different food sources. J. Ins. Physiol. 49, 307–313. doi: 10.1016/S0022-1910(03)00004-0
Silva, A., Rodrigues, A., Bacci, M., Pagnocca, F., and Bueno, O. C. (2006). Susceptibility of the ant-cultivated fungus Leucoagaricus gongylophorus (Agaricales: Basidiomycota) towards microfungi. Mycopathologia 162, 115–119.
Solomon, S. E., Rabeling, C., Sosa-Calvo, J., Lopes, C. T., Rodrigues, A., Vasconcelos, H. L., et al. (2019). The molecular phylogenetics of Trachymyrmex Forel ants and their fungal cultivars provide insights into the origin and coevolutionary history of ‘higher-attine’ ant agriculture. Syst. Entomol. 44, 939–956. doi: 10.1111/syen.12370
Suen, G., Scott, J. J., Aylward, F. O., Adams, S. M., Tringe, S. G., Pinto-Tomás, A. A., et al. (2010). An insect herbivore microbiome with high plant biomass-degrading capacity. PLoS Genet. 6:e1001129. doi: 10.1371/journal.pgen.1001129
Taerum, S. J., Cafaro, M. J., Little, A. E. F., Schultz, T. R., and Currie, C. R. (2007). Low host–pathogen specificity in the leaf-cutting ant–microbe symbiosis. Proc. R. Soc. B 274, 1971–1978. doi: 10.1098/rspb.2007.0431
Thompson, J. N. (1986). Constraints on arms races in coevolution. Trends Ecol. Evol. 1, 105–107. doi: 10.1016/0169-5347(86)90036-90034
Vale, P. F., Fenton, A., and Brown, S. P. (2014). Limiting damage during infection: lessons from infection tolerance for novel therapeutics. PLoS Biol. 12:e1001769. doi: 10.1371/journal.pbio.1001769
Varanda-Haifig, S. S., Albarici, T. R., Nunes, P. H., Haifig, I., Vieira, P. C., and Rodrigues, A. (2017). Nature of the interactions between hypocrealean fungi and the mutualistic fungus of leaf-cutter ants. Antonie Van Leeuwenhoek 110, 593–605. doi: 10.1007/s10482-016-0826-y
Wakelin, D. (1978). “Genetic control of susceptibility and resistance to parasitic infection,” in Advances in Parasitology, eds W. H. R. Lumsden, R. Muller, and J. R. Baker (Cambridge, MA: Academic Press), 219–308. doi: 10.1016/S0065-308X(08)60575-8
Wallace, D. E. E., Asensio, J. G. V., and Tomás, A. A. P. (2014). Correlation between virulence and genetic structure of Escovopsis strains from leaf-cutting ant colonies in Costa Rica. Microbiology 160, 1727–1736. doi: 10.1099/mic.0.073593-0
Wang, Y., Mueller, U. G., and Clardy, J. O. N. (1999). Antifungal diketopiperazines from symbiotic fungus of fungus-growing ant Cyphomyrmex minutus. J. Chem. Ecol. 25, 935–941.
Wilson, E. O. (1980). Caste and division of labor in leaf-cutter ants (Hymenoptera: Formicidae: Atta). Behav. Ecol. Sociobiol. 7, 157–165. doi: 10.1007/BF00299521
Keywords: host-pathogen interactions, opportunistic infections, pathogenesis, commensals, dysbiosis, host resistance, colony defenses
Citation: Jiménez-Gómez I, Barcoto MO, Montoya QV, Goes AC, Monteiro LSVE, Bueno OC and Rodrigues A (2021) Host Susceptibility Modulates Escovopsis Pathogenic Potential in the Fungiculture of Higher Attine Ants. Front. Microbiol. 12:673444. doi: 10.3389/fmicb.2021.673444
Received: 27 February 2021; Accepted: 14 May 2021;
Published: 14 June 2021.
Edited by:
Takema Fukatsu, National Institute of Advanced Industrial Science and Technology (AIST), JapanCopyright © 2021 Jiménez-Gómez, Barcoto, Montoya, Goes, Monteiro, Bueno and Rodrigues. This is an open-access article distributed under the terms of the Creative Commons Attribution License (CC BY). The use, distribution or reproduction in other forums is permitted, provided the original author(s) and the copyright owner(s) are credited and that the original publication in this journal is cited, in accordance with accepted academic practice. No use, distribution or reproduction is permitted which does not comply with these terms.
*Correspondence: Irina Jiménez-Gómez, irinajimenez1987@gmail.com; Mariana O. Barcoto, m.barcoto@unesp.br; Andre Rodrigues, andre.rodrigues@unesp.br
†These authors have contributed equally to this work