- 1Departamento de Ciências Biológicas, Universidade Estadual de Santa Cruz, Ilhéus, Brazil
- 2Departamento de Genética, Instituto de Ciências Biológicas, Universidade Federal de Minas Gerais, Belo Horizonte, Brazil
- 3Instituto de Biologia, Universidade Federal da Bahia, Salvador, Brazil
- 4Centro de Pesquisas do Cacau, Comissão Executivo do Plano da Lavoura Cacaueira, CEPEC/CEPLAC, Rodovia Ilhéus-Itabuna, Ilhéus, Brazil
- 5Instituto de Cultivos Tropicales, Tarapoto, Peru
- 6Universidad Nacional Autónoma de Alto Amazonas, Yurimaguas, Peru
Introduction: Theobroma cacao, the cocoa tree, is a target for pathogens, such as fungi from the genera Phytophthora, Moniliophthora, Colletotrichum, Ceratocystis, among others. Some cacao pathogens are restricted to specific regions of the world, such as the Cacao swollen shoot virus (CSSV) in West African countries, while others are expanding geographically, such as Moniliophthora roreri in the Americas. M. roreri is one of the most threatening cacao pathogens since it directly attacks the cacao pods driving a significant reduction in production, and therefore economic losses. Despite its importance, the knowledge about the microenvironment of this pathogen and the cocoa pods is still poorly characterized.
Methods: Herein we performed RNA sequencing of spores in differential stages of culture in a medium supplemented with cacao pod extract and mycelium collected of the susceptible variety ICT 7121 naturally infected by the pathogen to evaluate the diversity and transcriptional activity of microorganisms associated with the in vitro sporulation of M. roreri.
Results: Our data revealed a great variety of fungi and bacteria associated with M. roreri, with an exceptional diversity of individuals from the genus Trichoderma sp. Interestingly, the dynamics of microorganisms from different kingdoms varied proportionally, suggesting they are somehow affected by M. roreri culture time. We also identified three sequences similar to viral genomes from the Narnaviridae family, posteriorly confirmed by phylogenetic analysis as members of the genus Narnavirus. Screening of M. roreri public datasets indicated the virus sequences circulating in samples from Ecuador, suggesting a wide spread of these elements. Of note, we did not identify traces of the viral sequences in the M. roreri genome or DNA sequencing, restricting the possibility of these sequences representing endogenized elements.
Discussion: To the best of our knowledge, this is the first report of viruses infecting the fungus of the genus Moniliophthora and only the third description of viruses that are able to parasite elements from the Marasmiaceae family.
1. Introduction
Theobroma cacao L. (Malvaceae) is a tropical plant native to the Amazon, which grows naturally in the shade of tropical forests (Motamayor et al., 2002; Garcia et al., 2018). The genus Theobroma is constituted of 22 species, of which, the ones of economic importance are Theobroma cacao L. and Theobroma grandiflorum as they provide the raw material for chocolate production and other food industries (Bartley, 2005). In addition to many health benefits (Franco et al., 2013), the flavonoids in cocoa enter and build up in the parts of the brain responsible for memory and learning (Shahanas et al., 2019), consuming cocoa-derived products may also activate modifications in redox-sensitive signaling pathways that control gene expression and immunological response (Katz et al., 2011). For this reason, cocoa is classified as one of the most important commodities worldwide responsible for improving the economic livelihoods of farmers (Osorio-Guarín et al., 2020).
Chocolate has great demand and acceptance globally, however, its supply depends on the cultivation of cacao in favorable climatic conditions and low incidence of pests and diseases (Dantas Neto et al., 2005; Evans et al., 2013). Phytopathogenic fungi are one of the primary disease agents that attack cocoa plantations in tropical countries (Dos Santos et al., 2017). One example is frost pod rot (FPR), caused by Moniliophthora roreri, a hemibiotrophic basidiomycete belonging to the Marasmiaceae family (Aime and Phillips-Mora, 2005). This species is usually found infecting fruits of the plant genera Herrania and Theobroma (Bailey et al., 2018), impacting especially cocoa beans that can lead to yield losses of up to 80% (Hidalgo et al., 2003; Sánchez et al., 2012). In South American countries, the witches’ broom disease of cacao (WBD) caused by the fungi Moniliophthora perniciosa figure as one of the major threats to cocoa production that results in significant yield and economic losses (Marelli et al., 2009; Teixeira et al., 2015), ranging from 50 to 90% in affected regions (Meinhardt et al., 2008). Indeed, WBD has caused significant crop losses, especially in Brazil, where the yield of cacao dropped by more than 70% in the 10 years after the disease first appeared (Teixeira et al., 2014).
The origin of M. roreri occurred in Colombia, and then migrated to Ecuador and spread to other regions, such as Venezuela, Peru, and Central American countries (Phillips-Mora et al., 2007). The last report of the presence of the disease in an invasive profile had been in Jamaica (Johnson et al., 2017). Of note, the first outbreak of the disease in Brazil was recently described in the city of Cruzeiro do Sul (Acre state), with symptomatic plants identified, and, posteriorly, the presence of the fungi pathogen validated by the Federal Laboratory for Agricultural Defense – Goiânia (AGROLINK, 2021).
Other fungal pathogens also cause losses in cocoa. For example, the species Phytophthora megakarya in West Africa presents a threat to the crop in other regions due to its high virulence (Marelli et al., 2019). The fungus Ceratocystis cacaofunesta is one of the most aggressive pathogens impacting cocoa production in different countries, leading to the destruction of large numbers of Theobroma crops (Mora-Ocampo et al., 2021). Anthracnose, a disease caused by Colletotrichum spp., is a limiting factor in production and the causal agent of this disease has been reported in different countries, as in Ghana an anthracnose outbreak covered a cultivated area of 248.47 hectares, leading to losses (Asare et al., 2021), or in Brazil, in which Colletotrichum aeschynomenes was identified in October 2016 colonizing cocoa plants (Nascimento et al., 2019). Moreover, many cocoa-producing countries in West Africa, have been infected by viral diseases such as cocoa swollen stem disease (CSSD) caused by the Cocoa swollen shoot virus (CSSV; Abrokwah et al., 2016).
Due to the number of pathogens described infecting T. cacao, many studies have proposed to investigate the microbiota associated with the infected plant as well as to assess the potential of endophytes as agents for biological control of these pathogenic agents. A previous study in the Equatorial Amazon characterized the microbiota of cacao plants based on morphological criteria identifying several species from the genera Cyliindrocladium, Dichobotrys, Moniliophthora, Colletotrichum, and Phytophthora (Carrera-Sánchez et al., 2016). In addition, a study using morphological characteristics and ribosomal DNA sequencing focused on the branch part of cacao plants also revealed a great diversity of endophytic fungi by including the presence of species from the genera Acremonium, Blastomyces, Botryosphaeria, Cladosporium, Colletotrichum, Cordyceps, Diaporthe, Fusarium, Geotrichum, Gibberella, Gliocladium, Lasiodiplodia, Monilochoetes, Nectria, Pestalotiopsis, Phomopsis, Pleurotus, Pseudofusarium, Rhizopycnis, Syncephalastrum, Trichoderma, Verticillium, and Xylaria (Rubini et al., 2005). Several endophyte organisms were recorded directly in the field of healthy tree trunks and pods, and more than 40 genera were identified and recorded mainly representing anamorphs of Hypocreales in the genera Acremonium, Clonostachys, and Trichoderma (Evans et al., 2003).
The advent of High-Throughput Sequencing (HTS) and RNA sequencing (RNA-seq) technologies have revolutionized the study of transcriptionally active elements and enabled the assessment of hidden microbial diversity in terms of different environmental parameters (Colgan et al., 2017; Saminathan et al., 2018). Omics sciences have provided a more detailed view of microbial interactions (Aguiar-Pulido et al., 2016). For instance, metatranscriptomics allows access to both transcriptional active community members and the mapping of metabolic pathways (Urich et al., 2008). Moreover, the Omics studies have revealed important information about microbial diversity, and interpreting these interactions is fundamental for developing sustainable agricultural practices (Priya et al., 2021).
In our study, RNA deep sequencing was applied to identify and characterize the microbiota dynamics associated with spores of M. roreri in differential culture times and mycelium using medium supplemented with cacao extract collected from plants naturally infected by the pathogen. Our metagenomics approach revealed higher diversity of species related to fungal and bacterial kingdoms, in special to Trichoderma fungi that have been described with potential for biological control of the different diseases affecting the crop. Unexpectedly, we also identified viral species belonging to the Narnaviridae family likely infecting M. roreri. This is the first report of viral infection in this species. Altogether, our results provided important data on the understanding of microorganism dynamics during frosty pod rot infection in T. cacao and revealed new species that can play a role in fungi-plant interactions.
2. Materials and methods
2.1. Spore and mycelium production
The spores and vegetative mycelium of Moniliophthora roreri were obtained from naturally infected cocoa fruits of the susceptible cocoa variety ICT 7121 at the Instituto de Cultivos Tropicales (ICT) located in Tarapoto City, Peru in May 2015 through a partnership between Brazilian and Peruvian institutions. Four-month-old diseased cocoa pods affected by frosty pod rot disease, showing necrotic spots and white pseudostroma were harvested, washed with running water using a brush to eliminate the mycelium, and then immersed in a 2% sodium hypochlorite solution for 5 min. Then, they were dried with a paper towel, cut into two-cm thick slices, transversal to the longitudinal axis of the fruit, placed on the surface of Petri dishes, and kept in a humid chamber for three to 4 days at 21–25°C for growth of the vegetative mycelium (Supplementary Figures 1A, B). After cultivation, the mycelium was carefully removed by scraping the surface of the slices with the aid of a sterile scalpel (Supplementary Figure 1C). The mycelial mass was washed twice with 10% Trichloroacetic Acid (TCA) in Acetone. Two milliliters of RNAlater were then added to the dried pellet for further extraction of total RNA. An aliquot of this material was inoculated into a medium, as well as a positive control, in order to show that the chemical treatments performed made the spores non-viable. The fresh spores were inoculated on a medium containing cocoa broth after incubating the cocoa fruit slices for 5–8 days (Supplementary Figure 1D). For the preparation of the culture medium, 250 g of healthy susceptible variety ICT 7121 cocoa fruit were used, autoclaved in 1 L of distilled water. Then, the broth was strained, and 0.15% agar was added. After cooling, the cocoa agar medium was plated on Petri dishes. A total of 20 Petri dishes were used to inoculate 1 ml of M. roreri spore suspension at a concentration of 4.2 × 106 spores/ML for the times 8, 16, 24, and 48 h after inoculation (hai). The ungerminated dry spores were collected using a fine brush and a beaker with 100 ml of autoclaved water containing streptomycin at a concentration of 0.01%. This solution was homogenized with 0.01% Tween 80, then filtered through sterile gauze. The spores were counted in a Neubauer chamber. Then, the suspension was centrifuged to obtain the spore mass, which was washed in (TCA) 10% in Acetone twice, added 2 ml of RNAlater (Fisher Scientific, United States) to the dried pellet for further extraction of total RNA.
2.2. Total RNA extraction and quantification
After removal of the RNAlater, the dried inactive mycelium and spore pellets at inoculated times 0 (uninoculated), 8, 16, 24, and 48 hai were macerated using liquid nitrogen. For each sample, dry weights were obtained, and the samples were stored at -80°C. Total RNA was extracted with the ZR Plant RNA MiniPrepTM kit (Zymo Research, United States) following the manufacturer’s instructions. Total RNA was quantified by Qubit fluorimeter (Invitrogen, United States) using Qubit® dsRNA HS/BR kits and Nanodrop 2000c (Thermo Scientific, United States; Supplementary Table 1).
2.3. Library construction and sequencing
The messenger RNA (mRNA) libraries were constructed using the TruSeq RNA® v2 Low Sample (LS) kit (Illumina, United States) according to the manufacturer’s instructions. The purity level and the size of the fragments obtained from the mRNA libraries of the spores at different germination times (0, 8, 16, and 48 hai) and of the mycelium were checked on agarose gel 3%, using DNA markers of 50 bp (New England/Biolabs, United States), 100 bp (Gene Ruler/Fermentas, United States). Absolute quantification of the libraries was performed using the Kapa Library Quantification ABI Prism® qPCR Mix kit (Kapa Biosystems, United States) according to the manufacturer’s instructions. A pool of the samples at a concentration of 15 pM and 5% of the PhiX control at the same concentration was used for each sequencing run. The sequencing was outlined as follows: (i) sequencing only spores time 0 h after inoculation; (ii) sequencing mycelium and spore pool 48 hai; (iii) sequencing spore pool with 8 hai and spores with 16 hai, only one sequencing kit for each one of these outlines. The sequencing was performed in the MiSeq System equipment (Illumina, United States) located in the Center of Biotechnology and Genetics/UESC (CBG) using the MiSeq® Reagent Kit v3 (Illumina, United States) of 150 cycles. The libraries produced in our study were deposited in the NCBI SRA database under Project accession number: PRJNA854689.
2.4. Library processing
The obtained raw reads (reads size ranging from 35 to 76 bp) were subjected to a pre-processing step. Initially, reads with quality equal to or greater than Phred 30 and size greater than 20 bp were selected with the Trimmomatic 0.33 tool (Bolger et al., 2014). The remaining reads were used in a quality analysis with the FASTQC tool (Andrews, 2017). The filtered sequences were then mapped against the reference genomes of the plant T. cacao and the fungus M. roreri using the Bowtie2 tool (Langmead and Salzberg, 2012) to remove sequences from these organisms. The unmapped reads were used for the subsequent analyses. The reference genomes of Theobroma cacao and Moniliophthora roreri were downloaded from the NCBI GenBank database using the accession numbers GCA_000403535 and GCA_000488995.1, respectively. All analyses were performed using the Galaxy Bioinformatics platform (Afgan et al., 2018).1 The pre-processed reads were used in the subsequent analyses.
2.5. Metagenomics analysis
The pre-processed reads were used for contig assembly with the Trinity tool [Trinity de novo assembly of RNA-Seq data (Galaxy version 2.9.1)] using default parameters (Grabherr et al., 2011). The assembled contigs were evaluated on the Kaiju web server2 with the parameters (e-value <0.001) by searching the NCBI BLAST nr + euk − non-redundant protein database. Libraries that showed viral hits were subjected to sequence similarity analysis with Diamond (Buchfink et al., 2015) which returned multiple fragments for each library per condition that were subsequently reassembled with the CAP3 tool (Huang and Madan, 1999). The analysis of abundance at kingdom, genera, and species level was based on taxonomical classification by Kaiju which only elements showing abundance equal or greater than five were considered. Transcripts assigned to the species M. roreri and M. perniciosa were also removed from these analyzes. Species and genera with less than five transcripts are listed in Supplementary Data 1. The viral sequences identified in our study were deposited in NCBI GenBank database under the accession numbers: ON210269, ON210270, and ON210271.
2.6. Temporal analyses of beta diversity
In order to analyze changes in the assemblage composition between different times we calculated temporal beta diversity (Baselga et al., 2015) using the function “beta.temp” from “betapart” R package (Baselga et al., 2021) in program R (R Core Team, 2021). Temporal beta diversity uses a similar concept of spatial beta diversity, allowing the partitioning of beta diversity into turnover and nestedness components. However, instead of assessing how composition changes across a set of sites, it assesses changes on each site between different times (Baselga, 2010; Baselga et al., 2015). Because we have five time points (spores 0, 8, 16, 48 hai, and Mycelium), we calculated beta-diversity between each time and its subsequent time, which allowed us to compare the composition of assemblages across all pairs of successive times of infection. Beta diversity was calculated using Jaccard dissimilarity.
2.7. Phylogenetic analysis
A dataset containing the viral contigs assembled in our study and public protein sequences related to Mitovirus, Narnavirus, and Ourmiavirus was constructed and aligned using the MAFFT program (Katoh et al., 2019). The best evolutionary protein model was selected using ModelTest, considering Akaike’s information criterion (Akaike, 1998). Maximum likelihood inference was built in IqTree using 1,000 bootstrap replicates (Nguyen et al., 2015). The tree was rooted considering Escherichia virus as an external group (accession number: NP_040755) and edited in Figtree.3
2.8. Analyses of RNA and DNA sequencing public data from Moniliophthora roreri
Three publicly available deep sequencing libraries from M. roreri were downloaded from the SRA database. Two RNA-seq libraries (SRR1036616 and SRR1034656) contained mixed pathogen-infected plant material (M. roreri and T. cacao), derived from an M. roreri clone (MCA2977) isolated from the state of Los Rios, Ecuador (Meinhardt et al., 2014). The third library (SRR8453395), prepared from genomic DNA, was derived from M. roreri CPMRT01 isolate identified in T. cacao plants in Tabasco, Mexico (Hipólito-Romero et al., 2020). All three libraries were used to investigate the presence and abundance of viral sequences detected in our library using the Kallisto tool (Bray et al., 2016).
3. Results
3.1. De novo transcriptome assembly
The total RNA sequencing produced over 85 million reads distributed among the five libraries (conditions). The number of raw reads ranged from 12,384,924 in the mycelium condition to 25,840,512 in spores 0 hai (uninoculated; Supplementary Table 1). After pre-processing, more than 99% of the sequences were kept, supporting the quality of our RNA deep sequencing. Pre-processed sequences were submitted to de novo assembly with Trinity, producing a total of 169,074 transcripts. The number of transcripts assembled ranged from 20,879 in Mycelium to 65,605 in spores 48 hai, with mean varying from 508 to 780 nt and N50 between 604 to 1,088 nt (Supplementary Table 1).
3.2. Diversity of bacteria and fungi associated with Moniliophthora roreri infection in cocoa pods
The assembled transcripts were classified using sequence-similarity searches according to their closest relative in public databases. From the total 169,074 (100%) transcripts, 149,975 (88.17%) were classified at least at Kingdom level while 19,099 (11.24%) were unassigned. We observed the highest percentage of classified transcripts in the library of spores 8 hai. Library derived from Spores 48 hai showed the highest number of transcripts without taxonomic assignment (Figure 1A).
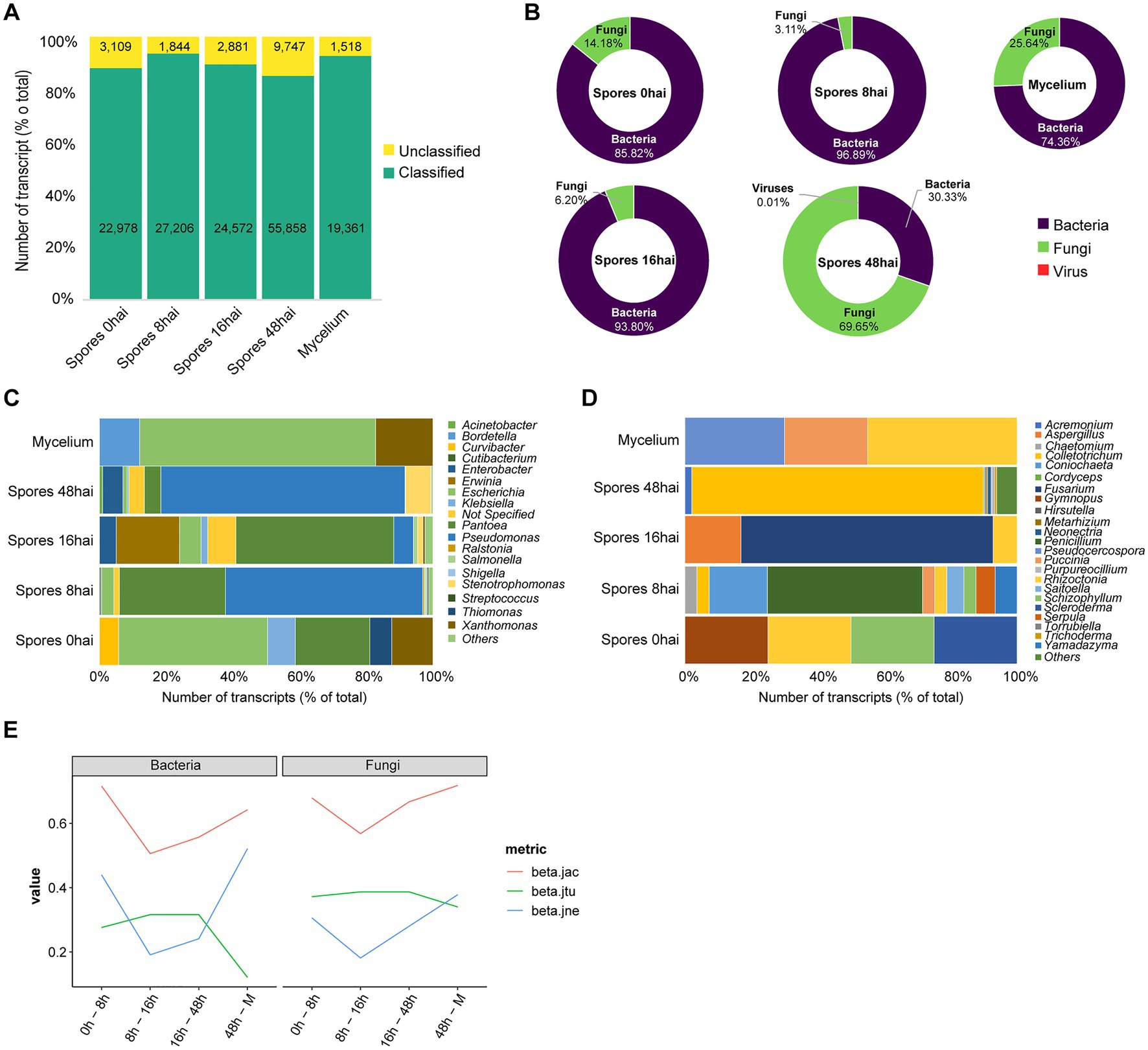
Figure 1. Metagenomics analysis of Theobroma cacao pods infected by Moniliophthora roreri. (A) Overview of taxonomic assignment of assembled transcripts by condition. (B) Abundance of fungi, bacteria and virus in the samples. Diversity of bacteria (C) and fungi (D) by genera. (E) Temporal changes in microbiota of T. cacao fruits along M. roreri infection time points. beta.jtu, beta.jne and beta.jac, represents the partitioning of beta diversity into turnover, nestedness and jackniffe, respectively. hai: hours after inoculation.
3.2.1. Bacterial diversity
From the 169,074 transcripts that did not match Moniliophthora species, 15,365 sequences could be assigned to bacterial species distributed in the libraries: spores 0 hai (121 transcripts), spores 8 hai (4,270 transcripts), spores 16 hai (1,255 transcripts), spores 48 hai (9,661 transcripts), and mycelium (58 transcripts; Figure 1B). The genus Pseudomonas had the highest number of elements assigned in all sequenced libraries with a median of 1933.8 transcripts per library (Figure 1C). Pseudomonas is one of the most ubiquitous bacterial genera and has been isolated worldwide from different environments, such as clinical, plants, fungi, and animal samples (Peix et al., 2009). Within Pseudomonas genera, the most abundant species were Pseudomonas parafulva (2,531 transcripts), Pseudomonas sp. Bc-h (1,188 transcripts), Pseudomonas sp. NFR16 (1,019 transcripts), Pseudomonas putida (928 transcripts), and Pseudomonas abietaniphila (878 transcripts). Other species that ranked in the top 10 were Pantoea dispersa (1,761 transcripts), Stenotrophomonas maltophilia (671 transcripts), Enterobacter cancerogenus (627 transcripts), Escherichia coli (456 transcripts), and Type-E symbiont of Plautia stali (431 transcripts).
Other genera also showed a large number of transcripts, such as Pantoea (2,453 transcripts), Enterobacter (663 transcripts), Acinetobacter (100 transcripts), Stenotrophomonas (745 transcripts), Escherichia (456 transcripts), Erwinia (250 transcripts), Klebsiella (86 transcripts), Xanthomonas (63 transcripts), and Salmonella (54 transcripts). The genus Escherichia was present in all five libraries. The Pantoea and Xanthomonas are present in four libraries, and Pseudomonas are present in three of the Spore conditions (Figure 1C). We noticed a clear change in the bacterial community between Spores and Mycelium, with some genera being condition-specific while others showed high prevalence among all conditions. Four different strains of the bacteria Pantoea dispersa, which had 1,761 transcripts assigned, have been described as inhibiting the mycelium growth of the fungus Ceratocytis fimbriata causing black rot in sweet potato, spore germination, as well as altering the morphology of fungal hyphae thus having biological control potential (Jiang et al., 2019).
3.2.2. Fungal diversity
Fungi was the most represented kingdom identified in cocoa pod microbiota. A total of 22,444 (68.05%) transcripts distributed between spores 0 hai (20), spores 8 hai (137), spores 16 hai (83), spores 48 hai (22,184), and mycelium (20) conditions were identified (Figure 1D). Analyzing the diversity at the genera level, we can highlight Colletotrichum (1924 transcripts), Fusarium (699 transcripts), Acremonium (440 transcripts), Neonectria (229 transcripts), Purpureocillium (129 transcripts), Trichoderma (129 transcripts), Metarhizium (102 transcripts), Hirsutella (89 transcripts), Cordyceps (88 transcripts), and Torrubiella (86 transcripts). Regarding fungi abundance, we note an unexpected abundance of transcripts derived from Colletotrichum elements in the library from Spores 48 hai. Some other genera, such as Rhizoctonia, were identified in three libraries (pores 0, 8, and 16 hai). On the other hand, many genera were represented only in specific conditions, such as Scleroderma (spores 0 hai), and Yamadazyma (spores 8 hai; Figure 1D).
At the species level, the most abundant fungal species was Colletotrichum gloeosporioides with 18,116 transcripts, followed by Acremonium chrysogenum with 440. We also noticed the presence of many species from the genus Trichoderma. Indeed, the species Trichoderma virens was present in all libraries while the library constructed from spores 48 hai showed the highest diversity of members from this genus, presenting transcripts from T. reesei, T. parareesei, T. harzianum, T. guizhouense, T. gamsii, and T. atrovirie species. In the same libraries, we also identified sequences related to the species Beauveria bassiana (11 assigned transcripts), another species from the Hypocreales order that is also considered endophytic.
3.3. Temporal changes in fungal and bacterial diversity
In order to assess the changes in microbiota composition in T. cacao pods according to M. roreri time of inoculation, we calculated the changes of beta diversity along the different times of infection. We observed higher changes in spores 0 hai compared to spores 8 hai and spores 48 hai compared to Mycelium (Figure 1E). This difference is mainly driven by richness observed in spores 8 hai and spores 48 hai which is at least two times higher than the other conditions (Supplementary Table 2). Interestingly, in most cases, with few exceptions (bacterial species from spores 0 hai to spores 8 hai and spores 48 hai to mycelium and fungal species from spores 48 hai to mycelium) the changes in the community structure were dominated by species turnover (i.e., species replacement were larger than the loss/gain of species; Supplementary Tables 3, 4 and Supplementary Data 1). This profile is highlighted in the comparison between spores 0 hai and mycelium, where the ratio of beta-diversity (turnover/nestedness) is 0.79 and 0.89 for bacterial and fungal species, respectively. Of note, we observed that the changes observed were very similar for fungi and bacteria, showing a strong Pearson correlation (r: 0.95 p: 0.00003). This result suggests these species are somehow similarly affected by M. roreri time of infection.
3.4. Characterization of viral sequences associated to Moniliophthora roreri
Our metagenomics analyses using Kaiju’s webserver identified four contigs showing similarity to viral sequences in the library constructed from spores 48 hai. Two contigs were closely related to the viral species Ophiostoma mitovirus 5, that according to the International Committee on Taxonomy of Viruses (ICTV) belongs to the genus Mitovirus – Mitoviridae (Lefkowitz et al., 2018). We also identified one contig related to the Sanxia narna-like virus 1, which is an unclassified RNA virus (Schoch et al., 2020). Finally, one contig presented similarity to Pseudomonas phage PPpW-3, a DNA virus associated with bacteria from the family Myoviridae.
Since we had indicative signs of viral presence in the samples, we performed an extra step of virus identification using sequence similarity searches against the NR database using the Diamond tool. Using this strategy, we detected 30 transcripts showing similarity to viral sequences related to species from the Narnaviridae family, genus Narnavirus (Supplementary Table 5). After redundancy removal and contig extension step, three viral sequences were kept, Contig 1 (2,460 nt), Contig 2 (2,332 nt) and Contig 3 (3,606 nt). These sequences were further validated by BLASTx searches at NCBI website to guarantee the most updated version of sequence databases (Supplementary Tables 6–8; Altschul et al., 1997). Contigs 1, 2 and 3 showed similarity to Erysiphe necator associated narnavirus 4 (QHD64827.1), Magnaporthe oryzae narnavirus 1 (BCH36655.1), and Monilinia narnavirus H (QED42934.1), respectively. Structural annotation revealed that Contig 1 encodes to an open reading frame of 788 amino acids, Contig 2 (754 amino acids), and Contig 3 (1,085 amino acids; Figure 2A). Of note, the search for domains did not reveal any conserved region for any of the three putative viral genomes, which is common for elements from Narnavirus genus.
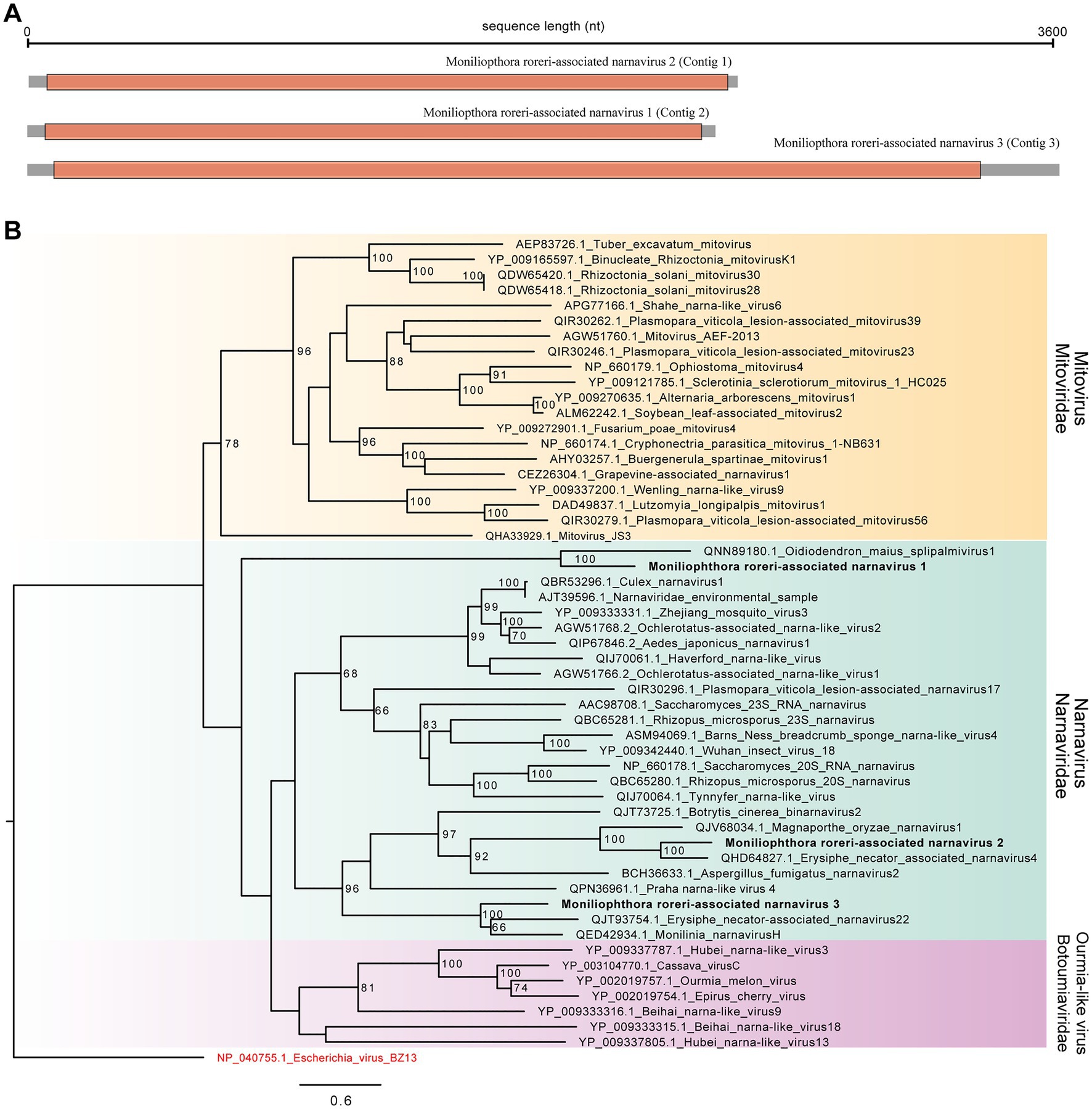
Figure 2. Characterizations of Moniliophthora roreri-associated viral sequences. (A) Genomic structure of M. roreri associated viruses. Gray square represents the whole viral segment while orange region indicates the large Open Read Frame. (B) Maximum likelihood tree constructed based on large viral ORF translated into protein. Viral sequences identified in the work are highlighted in bold. Bootstrap values larger than 60 are indicated in the tree.
3.5. Phylogenetic characterization of Moniliophthora roreri-associated viral sequences
In order to further characterize the putative viral sequences identified in our study, we performed phylogenetic analyses with closely related sequences present in public databases and related families indicated by ICTV, Mitoviridae and Botoumiaviridae. As observed in sequence similarity searches, the three viral transcripts clustered with different species of the Narnaviridae family. Contig 1 clustered with the species Erysiphe necator narnavirus 4 (QHD64827), while Contig 2 grouped with the species Oidiodendron maius splipalmivirus 1 (QNN89180), both showing 100 bootstrap replicates. Contig 3 formed a cluster with the species Erysiphe necator narnavirus 22 (QJT93754) and Monilinia narnavirus H (QED42934). According to our phylogenetic analysis, the contigs identified in our study represent new viral genomes related to the Narnaviridae family, specifically from Narnavirus genus. They were named Moniliophthora roreri-associated narnavirus 1 (Contig 2), Moniliophthora roreri-associated narnavirus 2 (Contig 1) and Moniliophthora roreri-associated narnavirus 3 (Contig 3) to reflect host origin (Figure 2B).
3.6. Presence of Moniliophthora roreri-associated viruses in public data
To evaluate the presence of the viral sequences found in the cocoa pods affected by frosty pod rot disease sequenced in our study, we investigated publicly available RNA deep-sequenced libraries derived from M. roreri. We were able to identify the presence of the Moniliophthora roreri-associated narnavirus 2 and Moniliophthora roreri-associated narnavirus 1 in two libraries from Los Rios, Ecuador submitted by the USDA, containing mixed material from 30 days and 60 days after inoculation of T. cacao pods with the M. roreri (Figure 3).
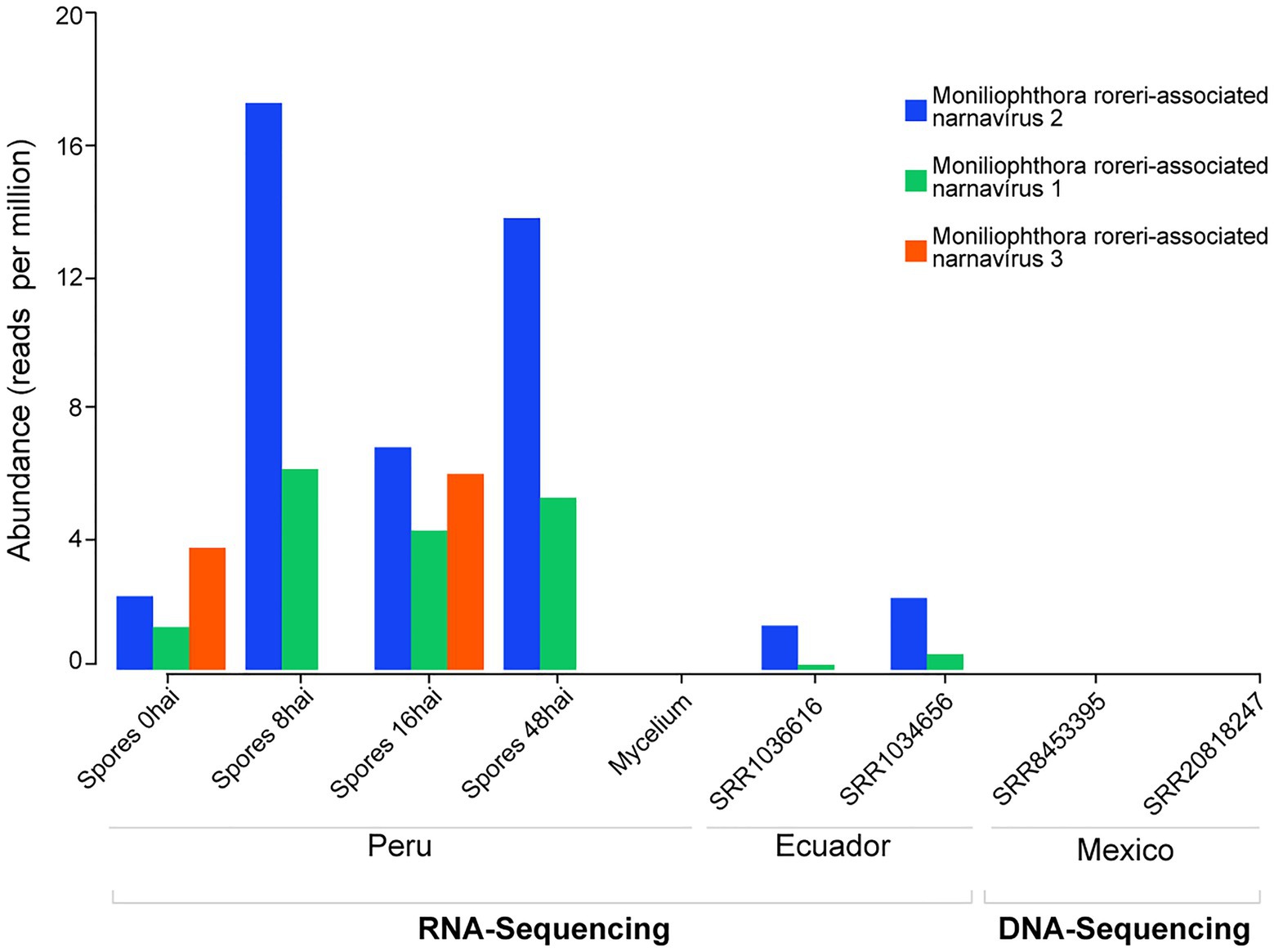
Figure 3. Presence of Moniliophthora roreri-associated viral sequences in public datasets. RNA and DNA deep sequencing libraries deposited at NCBI SRA databases from samples originated in different countries were investigated regarding the presence of reads derived from M. roreri-associated viral sequences.
To rule out the possibility of these sequences representing viral elements integrated into the genome of M. roreri we also searched fragments of M. roreri-associated viruses in the fungi genome and DNA sequencing libraries. As expected for RNA viruses that do not produce DNA intermediates in their replication cycle, we were not able to find signals of the viral sequences in the M. roreri genome or genomic libraries (Figure 3).
Since M. roreri and M. perniciosa are related and often occur in the same region, we also assessed the possible presence of the M. roreri-associated viruses in public RNA deep-sequenced libraries derived from the sister taxon. Therefore, we searched for the viruses in 10 samples of healthy seedlings and seedlings 30 days after M. perniciosa infection (Teixeira et al., 2014). However, we were not able to identify positive hits for the M. roreri-associated viruses identified in our study.
4. Discussion
The cacao tree (Theobroma cacao L.) is a crop of economic importance to many countries in the world. Nevertheless, its production and supply of almonds, which is the main source for chocolate manufacturing, has been hampered by unfavorable weather conditions (Wessel and Quist-Wessel, 2015) and pathogen attacks leading to economic losses and plantation abandonment. Therefore, an effort is necessary to address the problems associated with the control and management of different diseases that affect cocoa production to ensure the economic sustainability of producers (Adeniyi, 2019). Fungal diseases, and Moniliophthora species, in particular, remain a major constraint on cocoa production in the Americas and, if they exceed their current range, threaten to seriously damage the chocolate industry worldwide (McElroy et al., 2018), as in Panama, attacks by M. roreri and Phytophthora sp. cause losses of up to 80% of pods, affecting production (Krauss et al., 2006).
In our study, we performed an exploratory study to investigate the microbiota of spores at different times after inoculation and the mycelium of M. roreri using a RNA-seq approach. Our data revealed abundance and diversity of many fungal genera, such as Fusarium, Acremonium, Neonectria, Purpureocillium, Trichoderma, Metarhizium, Hirsutella, Cordyceps, Torrubiella, among others. Moreover, the bacterial genera with higher abundance in our study were Pseudomonas, Pantoea, Stenotrophomonas, Enterobacter, Escherichia, Erwinia, Acenitobacter, Klebsiella, Xanthomonas, Salmonella and others. A close look at the taxonomic profile of cocoa pods community also revealed the presence of important species such as those of the genus Colletotrichum, a genus that is composed of elements with an economic impact on crops, in addition to its importance as a model for the study the evolution of host specificity, speciation and reproductive behaviors (Baroncelli et al., 2016). The results found in our study were similar to other metagenomic studies published in the literature, for example, the study conducted by (Carrera-Sánchez et al., 2016), in which plant material infected by M. roreri was collected to analyze the microbiota associated with the disease. The results showed the presence of the genera Cylindrocladium, Dichobotrys, Moniliophthora, Colletotrichum, and Phytophthora. Another study focused on the assessment of cacao rhizosphere through metatranscriptomics revealed rhizobacteria being plant-growth promoters under drought conditions (Tamchek et al., 2019). Metagenomic analysis of the cocoa bean fermentation microbiome also identified a high percentage of bacteria, yeasts, and bacteriophages from the cocoa beans microbiome. The species Lactobacillus, Gluconacetobacter, Acetobacter, and Gluconobacter presented a greater dominance (Agyirifo et al., 2019). Another metagenomic sequencing in fermenting cacao pods revealed 97 genera, with Acetobacter, Komagataeibacter, Limosilactobacillus, Liquorilactobacillus, Lactiplantibacillus, Leuconostoc, Paucilactobacillus, Hanseniaspora, and Saccharomyces dominating (Verce et al., 2021). Endophytic bacterial species such as Pseudomonas aeruginosa, Chryseobacterium proteolyticum were isolated from healthy cocoa tissues, such as leaves and fruits, and both species showed a reduction of pod injury in disease caused by the fungal pathogen Phytophthora palmivora (Alsultan et al., 2019).
Cocoa pods harbor a diversity of microorganisms, some of them involved in the fermentation process such as species of the genus acetobacter, Komagataeibacter, Erwinia, Pantoea, Tatumella (Garcia-Armisen et al., 2010; Verce et al., 2021) and lactic acid bacteria such as Lactobacillus present in the cocoa fermentation microbiome with diverse functions (Serra et al., 2019). Other bacterial species can act as biological controllers of plant pathogens such as Pseudomonas spp., although the ecology of these populations is not fully understood (McSpadden Gardener, 2007), species of the genus Streptomyces are known to live endophytically in cocoa pods and seeds, promoting growth and favoring plant health (Tchinda et al., 2016).
A plentiful source of microorganisms that can, both directly and indirectly, support plant growth, defense, and development is found in the endophytic niches of plants (Hanada et al., 2010). Endophytic bacteria colonize the roots of cocoa trees efficiently and these species have promising results in inhibiting fungal growth (Alves-Júnior et al., 2021). Endophytic fungi develop most or all of their life cycle colonizing the host plant tissues, without causing evident damage, and some have benefits against the attack of phytopathogens (Sánchez-Fernández et al., 2013). Endophytic fungi with their wide range of biodiversity have provided useful insights for better knowledge of plant-fungi interaction and their role in host-associated microbiomes (Christian et al., 2015). Indeed, plants interact with a wide variety of fungal endophytes that reside in different tissues, sometimes contributing to plant growth and/or defense against biotic and abiotic stress (Gange et al., 2019). In healthy cocoa pods, 25 endophytic fungi were isolated and characterized morphologically with their reproductive structures. Furthermore, tests in vitro and in vivo using the endophytic fungi Aspergillus, Fusarium, and Ramichloridium, presented activity against P. palmivora in double culture, pod and seedling assays (Simamora et al., 2021). In our study the genera Fusarium were among the 10 most abundant, presenting many species that could play a role as biological controllers in T. cacao.
Necrotrophic, hemibiotrophic, latent or quiescent, and endophytic are general descriptions of the life modes of Colletotrichum species, with hemibiotrophic being the most prevalent (De Silva et al., 2017). The specie C. gloeosporioides can be considered endophytic and phytopathogenic. This specie mainly attacks young and soft cocoa leaves causing brown lesions surrounded by a characteristic light-yellow halo (Maximova et al., 2006). Anthracnose is a disease caused by Colletotrichum sp. that leads to yield reduction in many crops worldwide (da Silva et al., 2020). The genus is considered the eighth most important group of phytopathogenic fungi in the world, based on scientific and economic insights (Dean et al., 2012). Recently, there was a detection of C. gloeosporioides in native cashew species in Brazil (dos Santos et al., 2019). Two new species have been described by (Rojas et al., 2010) C. tropicale and C. ignotum and they are considered frequently asymptomatic in cocoa crops. In our study, we identified 14 species associated with the cocoa fruits: Colletotrichum chlorophyti, Colletotrichum fioriniae, Colletotrichum gloeosporioides, Colletotrichum graminicola, Colletotrichum higginsianum, Colletotrichum incanum, Colletotrichum nymphaeae, Colletotrichum orbiculare, Colletotrichum orchidophilum, Colletotrichum salicis, Colletotrichum siamense, Colletotrichum simmondsii, Colletotrichum sublineola, and Colletotrichum tofieldiae.
The second species with the highest number of transcripts assigned (440) was the filamentous fungus Acremonium chrysogenum (Ascomycota), a specie with industrial importance due to its ability to produce cephalosporin C (CPC), the main source for the production of different antibiotics in the industry (Hu and Zhu, 2016). The necrotrophic phytopathogen Neonectria ditíssima (Ascomycota) responsible for the European canker disease (EC) has 229 transcripts in our libraries, it is one of the most damaging apple diseases worldwide and has been recorded to be present in North and South American apple crops resulting in tree loss (Florez et al., 2020).
One of the 10 most abundant fungal genera in the microbiota was Trichoderma. Species such as T. theobromicola and T. paucisporum have been isolated from cocoa in South America, both inhibited the in vitro development of M. roreri (Samuels et al., 2006). Trichoderma species are common in soil and root ecosystems and are widely studied due to their great ability to produce metabolites with the potential to inhibit other microorganisms, parasitize other fungi, and compete with other microorganisms (Harman, 2006). Isolates of Trichoderma sp. species T. virens and T. harzianum have shown promise for the control of M. roreri (Reyes-Figueroa et al., 2016). Several isolates have been described as potential biological controls of moniliasis, such as Trichoderma isolates from different regions of Colombia, which have demonstrated mycelial growth antagonistic potential against strains of the fungus M. roreri (Suárez and Cabrales, 2008). A biocontrol strain using the fungus T. reesei (C2A) exhibited, in vitro, mycoparasitic activity, reducing 62% of the mycelial growth in Fusarium oxysporum (Gonzalez et al., 2020). These results reinforce the potential of strains of Trichoderma for the biological formulations to control the Moniliase disease (Leiva et al., 2020). Trichoderma harzianum Rifai from an infected cocoa pod is able to produce nonanoic (pelargonic) acid, which significantly reduces the spore germination of M. roreri in vitro (Aneja et al., 2005). The species T. viride can produce secondary metabolites (viridin and gliovirin) that have a synergistic effect for inhibiting the mycelial growth and conidium germination of fungal species Phythopthora, P. palmivora, and P. megakaria (Pakora et al., 2018). In our study, the following species were identified, T. virens, T. reesei, T. parareesei, T. harzianum, T. guizhouense, T. gamsii, and T. atroviride. According to the literature, some species found in our study have the potential for biological control (Ferreira and Musumeci, 2021; Chochocca et al., 2022), and others can test the effects they will produce on the fungus M. roreri in vitro. We also identified in the 48-h spore library, transcripts related to the species Beauveria bassiana (11 assigned transcripts). Beauveria bassiana is considered endophytic in nature (Amobonye et al., 2020). In addition to having a detrimental impact on insect survival, inoculation of B. bassiana into cowpea plants increased plant height, leaf count and dry mass (Pachoute et al., 2021).
In our study, we also evaluated viral diversity. There were three viral contigs assigned to the Narnaviridae by similarity search and phylogenetic analysis. All three contigs assembled in our study represented novel genomes. According to the ICTV, two species are described for this family: the Saccharomyces 20S RNA narnavirus and Saccharomyces 23S RNA narnavirus, which have genomic compositions of positive-sense single-stranded RNAs (ssRNA viruses (+); Lefkowitz et al., 2018). A characteristic of the members of this family is that they contain simpler genomes than any RNA virus, ranging from 2.3 to 3.6 kb coding only a single polypeptide that has an RNA-dependent RNA polymerase domain (Hillman and Cai, 2013). Members of this family have been reported as fungal viruses or mycoviruses, being found in a variety of fungal species associated with asymptomatic infections (Ghabrial and Suzuki, 2008). Additionally, they have been found in pathogenic fungi, such as Rhizoctonia solani, Magnaporthe oryzae, Cercospora beticola (Abdoulaye et al., 2019; Liu et al., 2020; Li et al., 2021) and the ectomycorrhizal fungus Geopora sumneriana (Sahin and Akata, 2019).
Metagenomic approaches can be useful for detecting and identifying new mycoviruses (Son et al., 2015). These methods are rapidly increasing viral identification and providing evidence of their high abundance and taxonomic complexity (García-Pedrajas et al., 2019). Mycovirus infections are usually cryptic (asymptomatic), but investigations focus on the potential hypovirulence they can cause in the host fungus, a process that can be researched in the context of providing sustainable biological control of fungal diseases (Abdoulaye et al., 2019). For example, the fungus Colletotrichum sp. is one of the most economically important phytopathogens and can be infected by many different species of viruses presenting modifications in its development (Casas et al., 2021).
The three contigs assembled in our study can be new viral genomes with similarities to the Narnaviridae. In our phylogenetic tree, Contig 1 was next to the species Magnaporthe Oryzae Narnavirus 1, and was described in the rice blast fungus Magnaporthe oryzae (Lin et al., 2020). Contig 2 grouped with the species Oidiodendron maius splipalmivirus 1 (Sutela et al., 2020). Contig 3 formed a cluster with the species Erysiphe necator-associated narnavirus 22 and Monilinia narnavirus H. Our results demonstrate that viral diversity is unexplored, and many viral species can be detected and identified infecting fungal species. These viruses may also present function as biological control agents since they can reduce fungal growth. However, although we have considerable amount of data that indicated M. roreri as host, since we were not able to perform further experiments, we still uncertain if the viruses are specifically infecting M. roreri, other fungal species or multiple species within that microenvironment assessed. Therefore, they were named with the prefix “M. roreri-associated” viruses.
Each plant can present a unique microbiome. The associated microorganisms might colonize different plant tissues and their abundance will depend on the nutrient availability, the planting immune-response system, the competence, the associations between other microorganisms, among others. In our study, we explored the microbiota associated with the infection caused by M. roreri in cocoa and found a wide variety of fungal, bacterial, and viral species. Moreover, we describe the presence of three new viral genomes infecting M. roreri. Our results can help other studies investigate the role of microorganisms during infection of M. roreri in cocoa fruits.
Data availability statement
The datasets presented in this study can be found in online repositories. The names of the repository/repositories and accession number(s) can be found at: http://www.ncbi.nlm.nih.gov/sra, PRJNA854689.
Author contributions
EA: conceptualization, methodology, and supervision. KN, BR, NH, and PF: formal analysis. CP, KG, and EA-G: resources. BR, PF, and EA: writing of the original draft. BR, PF, CP, KG, LC, and EA: reviewing and editing. CP: funding acquisition. All authors contributed to the article and approved the submitted version.
Funding
This work was funded by the Coordenação de Aperfeiçoamento de Pessoal de Nível Superior (CAPES, Finance Code 001), Conselho Nacional de Desenvolvimento Científico e Tecnológico (CNPq #490655/2013-0, #303765/2019-4, and #403670/2020-9), Fundação de Apoio á Pesquisa do Estado da Bahia (Convênio 067/2013).
Acknowledgments
We would like to show our gratitude for the ideas and suggestions raised during the scientific discussions at Proteomics Group and Virus Bioinformatics Laboratory, and the Center of Biotechnology and Genetics.
Conflict of interest
The authors declare that the research was conducted in the absence of any commercial or financial relationships that could be construed as a potential conflict of interest.
Publisher’s note
All claims expressed in this article are solely those of the authors and do not necessarily represent those of their affiliated organizations, or those of the publisher, the editors and the reviewers. Any product that may be evaluated in this article, or claim that may be made by its manufacturer, is not guaranteed or endorsed by the publisher.
Supplementary material
The Supplementary material for this article can be found online at: https://www.frontiersin.org/articles/10.3389/fmicb.2022.1053562/full#supplementary-material
Footnotes
References
Abdoulaye, A. H., Foda, M. F., and Kotta-Loizou, I. (2019). Viruses infecting the plant pathogenic fungus rhizoctonia solani. Viruses 11:1113. doi: 10.3390/v11121113
Abrokwah, F., Dzahini-Obiatey, H., Galyuon, I., Osae-Awuku, F., and Muller, E. (2016). Geographical distribution of cacao swollen shoot virus molecular variability in Ghana. Plant Dis. 100, 2011–2017. doi: 10.1094/PDIS-01-16-0081-RE
Adeniyi, D. (2019). Diversity of cacao pathogens and impact on yield and global production. Theobroma Cacao – Deploying Science for Sustainability of Global Cocoa Economy. IntechOpen.
Afgan, E., Baker, D., Batut, B., van den Beek, M., Bouvier, D., Cech, M., et al. (2018). The galaxy platform for accessible, reproducible and collaborative biomedical analyses: 2018 update. Nucleic Acids Res. 46, W537–W544. doi: 10.1093/nar/gky379
AGROLINK (2021). Brasil detecta foco de praga do cacau e cupuaçu. Disponível em: https://www.agrolink.com.br/noticias/brasil-detecta-foco-de-praga-do-cacau-e-cupuacu_452707.html (Acessoem 18 November, 2021).
Aguiar-Pulido, V., Huang, W., Suarez-Ulloa, V., Cickovski, T., Mathee, K., and Narasimhan, G. (2016). Metagenomics, metatranscriptomics, and metabolomics approaches for microbiome analysis. Evol. Bioinformatics Online 12s1, 5–16. doi: 10.4137/EBO.S36436
Agyirifo, D. S., Wamalwa, M., Otwe, E. P., Galyuon, I., Runo, S., Takrama, J., et al. (2019). Metagenomics analysis of cocoa bean fermentation microbiome identifying species diversity and putative functional capabilities. Heliyon 5:e02170. doi: 10.1016/j.heliyon.2019.e02170
Aime, M. C., and Phillips-Mora, W. (2005). The causal agents of witches’ broom and frosty pod rot of cacao (chocolate, Theobroma cacao) form a new lineage of Marasmiaceae. Mycologia 97, 1012–1022. doi: 10.3852/mycologia.97.5.1012
Akaike, H. (1998). “Information theory and an extension of the maximum likelihood principle,” in Selected Papers of Hirotugu Akaike. eds. E. Parzen, K. Tanabe, and G. Kitagawa (New York, NY: Springer), 199–213.
Alsultan, W., Vadamalai, G., Khairulmazmi, A., Saud, H. M., Al-Sadi, A. M., Rashed, O., et al. (2019). Isolation, identification and characterization of endophytic bacteria antagonistic to Phytophthora palmivora causing black pod of cocoa in Malaysia. Eur. J. Plant Pathol. 155, 1077–1091. doi: 10.1007/s10658-019-01834-8
Altschul, S. F., Madden, T. L., Schäffer, A. A., Zhang, J., Zhang, Z., Miller, W., et al. (1997). Gapped BLAST and PSI-BLAST: a new generation of protein database search programs. Nucleic Acids Res. 25, 3389–3402. doi: 10.1093/nar/25.17.3389
Alves-Júnior, M., de Sousa, F. O., Silva, T. F., Albino, U. B., Garcia, M. G., de Moreira, S. M. C. O., et al. (2021). Functional and morphological analysis of isolates of phylloplane and rhizoplane endophytic bacteria interacting in different cocoa production systems in the Amazon. Curr. Res. Microb. Sci. 2:100039. doi: 10.1016/j.crmicr.2021.100039
Amobonye, A., Bhagwat, P., Pandey, A., Singh, S., and Pillai, S. (2020). Biotechnological potential of Beauveria bassiana as a source of novel biocatalysts and metabolites. Crit. Rev. Biotechnol. 40, 1019–1034. doi: 10.1080/07388551.2020.1805403
Aneja, M., Gianfagna, T. J., and Hebbar, P. K. (2005). Trichoderma harzianum produces nonanoic acid, an inhibitor of spore germination and mycelial growth of two cacao pathogens. Physiol. Mol. Plant Pathol. 67, 304–307. doi: 10.1016/j.pmpp.2006.05.002
Asare, E., Domfeh, O., Avicor, S., Pobee, P., Bukari, Y., and Amoako-Attah, I. (2021). Colletotrichum gloeosporioides s.l. causes an outbreak of anthracnose of cacao in Ghana. S. Afr. J. Plant Soil 38, 107–115. doi: 10.1080/02571862.2020.1863485
Bailey, B. A., Evans, H. C., Phillips-Mora, W., Ali, S. S., and Meinhardt, L. W. (2018). Moniliophthora roreri, causal agent of cacao frosty pod rot. Mol. Plant Pathol. 19, 1580–1594. doi: 10.1111/mpp.12648
Baroncelli, R., Amby, D. B., Zapparata, A., Sarrocco, S., Vannacci, G., Le Floch, G., et al. (2016). Gene family expansions and contractions are associated with host range in plant pathogens of the genus Colletotrichum. BMC Genomics 17:555. doi: 10.1186/s12864-016-2917-6
Bartley, B. G. D. (2005). Genetic diversity of cacao and its utilization. Genet. Divers. Cacao Its Utilizat. 1–341. doi: 10.1079/9780851996196.0000
Baselga, A. (2010). Partitioning the turnover and nestedness components of beta diversity. Glob. Ecol. Biogeogr. 19, 134–143. doi: 10.1111/j.1466-8238.2009.00490.x
Baselga, A., Bonthoux, S., and Balent, G. (2015). Temporal beta diversity of bird assemblages in agricultural landscapes: land cover change vs. stochastic processes. PLoS One 10:e0127913. doi: 10.1371/journal.pone.0127913
Baselga, A., Orme, D., Villeger, S., Bortoli, J. D., Leprieur, F., Logez, M., et al. (2021). Betapart: partitioning beta diversity into turnover and nestedness components. Version:1.5.4.
Bolger, A. M., Lohse, M., and Usadel, B. (2014). Trimmomatic: a flexible trimmer for illumina sequence data. Bioinformatics 30, 2114–2120. doi: 10.1093/bioinformatics/btu170
Bray, N. L., Pimentel, H., Melsted, P., and Pachter, L. (2016). Near-optimal probabilistic RNA-seq quantification. Nat. Biotechnol. 34, 525–527. doi: 10.1038/nbt.3519
Buchfink, B., Xie, C., and Huson, D. H. (2015). Fast and sensitive protein alignment using DIAMOND. Nat. Methods 12, 59–60. doi: 10.1038/nmeth.3176
Carrera-Sánchez, K., Herrera Isla, L., Díaz Castellanos, M., and Leiva-Mora, M. (2016). Micobiota asociada a frutos de cacao con síntomas de moniliasis en la amazonía ecuatoriana. Centro Agríc. 43, 48–54.
Casas, L. L., Azevedo, J. L., Almeida, L. N., Costa-Neto, P. Q., Bianco, R. A., and Pereira, J. O. (2021). Mycoviruses infecting Colletotrichum spp.: a comprehensive review. Braz. J. Biol. 83:e248975. doi: 10.1590/1519-6984.248975
Chochocca, R. R. S., Avila, E. G., Rojas, J. H. F., Suazo, J. M. A., Cruz, A. R. H. D. L., Mohamed, M. M. H., et al. (2022). Antifungal effect from Zingiber officinale, Aloe vera and Trichoderma sp. for control of Moniliophthora roreri in Theobroma cacao in Huánuco, Peru. Rev. Fac. Nac. Agron. Medellin. 75, 9823–9830. doi: 10.15446/rfnam.v75n1.95804
Christian, N., Whitaker, B. K., and Clay, K. (2015). Microbiomes: unifying animal and plant systems through the lens of community ecology theory. Front. Microbiol. 6:869. doi: 10.3389/fmicb.2015.00869
Colgan, A. M., Cameron, A. D., and Kröger, C. (2017). If it transcribes, we can sequence it: mining the complexities of host–pathogen–environment interactions using RNA-seq. Curr. Opin. Microbiol. 36, 37–46. doi: 10.1016/j.mib.2017.01.010
da Silva, L. L., Moreno, H. L. A., Correia, H. L. N., Santana, M. F., and de Queiroz, M. V. (2020). Colletotrichum: species complexes, lifestyle, and peculiarities of some sources of genetic variability. Appl. Microbiol. Biotechnol. 104, 1891–1904. doi: 10.1007/s00253-020-10363-y
Dantas Neto, A., Corrêa, R. X., Monteiro, W. R., Luz, E. D. M. N., Gramacho, K. P., and Lopes, U. V. (2005). Caracterização de uma população de cacaueiro para mapeamento de genes de resistência à vassoura-de-bruxa e podridão-parda. Fitopatol. Bras. 30, 380–386. doi: 10.1590/S0100-41582005000400007
De Silva, D. D., Crous, P. W., Ades, P. K., Hyde, K. D., and Taylor, P. W. J. (2017). Life styles of Colletotrichum species and implications for plant biosecurity. Fungal Biol. Rev. 31, 155–168. doi: 10.1016/j.fbr.2017.05.001
Dean, R., van Kan, J. A. L., Pretorius, Z. A., Hammond-Kosack, K. E., di Pietro, A., Spanu, P. D., et al. (2012). The top 10 fungal pathogens in molecular plant pathology. Mol. Plant Pathol. 13, 414–430. doi: 10.1111/j.1364-3703.2011.00783.x
dos Santos, G. R., Chagas, J. F. R., Rodrigues-Silva, N., Sarmento, R. A., Leão, E. U., da Silva, R. S., et al. (2019). Detection of Colletotrichum gloeosporioides in native cashew species in Brazil. Braz. J. Microbiol. 50, 899–903. doi: 10.1007/s42770-019-00142-x
Dos Santos, F. N., Tata, A., Belaz, K. R. A., Magalhães, D. M. A., Luz, E. D. M. N., and Eberlin, M. N. (2017). Major phytopathogens and strains from cocoa (Theobroma cacao L.) are differentiated by MALDI-MS lipid and/or peptide/protein profiles. Anal. Bioanal. Chem. 409, 1765–1777. doi: 10.1007/s00216-016-0133-5
Evans, H. C., Bezerra, J. L., and Barreto, R. W. (2013). Of mushrooms and chocolate trees: aetiology and phylogeny of witches’ broom and frosty pod diseases of cacao. Plant Pathol. 62, 728–740. doi: 10.1111/ppa.12010
Evans, H. C., Holmes, K. A., and Thomas, S. E. (2003). Endophytes and mycoparasites associated with an indigenous forest tree, Theobroma gileri, in Ecuador and a preliminary assessment of their potential as biocontrol agents of cocoa diseases. Mycol. Prog. 2, 149–160. doi: 10.1007/s11557-006-0053-4
Ferreira, F. V., and Musumeci, M. A. (2021). Trichoderma as biological control agent: scope and prospects to improve efficacy. World J. Microbiol. Biotechnol. 37:90. doi: 10.1007/s11274-021-03058-7
Florez, L. M., Scheper, R. W. A., Fisher, B. M., Sutherland, P. W., Templeton, M. D., and Bowen, J. K. (2020). Reference genes for gene expression analysis in the fungal pathogen Neonectria ditissima and their use demonstrating expression up-regulation of candidate virulence genes. PLoS One 15:e0238157. doi: 10.1371/journal.pone.0238157
Franco, R., Oñatibia-Astibia, A., and Martínez-Pinilla, E. (2013). Health benefits of methylxanthines in cacao and chocolate. Nutrients 5, 4159–4173. doi: 10.3390/nu5104159
Gange, A. C., Koricheva, J., Currie, A. F., Jaber, L. R., and Vidal, S. (2019). Meta-analysis of the role of entomopathogenic and unspecialized fungal endophytes as plant bodyguards. New Phytol. 223, 2002–2010. doi: 10.1111/nph.15859
Garcia, C., Marelli, J.-P., Motamayor, J. C., and Villela, C. (2018). “Somatic embryogenesis in Theobroma cacao L” in Plant Cell Culture Protocols. Vol. 1815. eds. V. M. Loyola-Vargas and N. Ochoa-Alejo (New York: Springer), 227–245.
Garcia-Armisen, T., Papalexandratou, Z., Hendryckx, H., Camu, N., Vrancken, G., De Vuyst, L., et al. (2010). Diversity of the total bacterial community associated with Ghanaian and Brazilian cocoa bean fermentation samples as revealed by a 16 S rRNA gene clone library. Appl. Microbiol. Biotechnol. 87, 2281–2292. doi: 10.1007/s00253-010-2698-9
García-Pedrajas, M. D., Cañizares, M. C., Sarmiento-Villamil, J. L., Jacquat, A. G., and Dambolena, J. S. (2019). Mycoviruses in biological control: from basic research to field implementation. Phytopathology 109, 1828–1839. doi: 10.1094/PHYTO-05-19-0166-RVW
Ghabrial, S. A., and Suzuki, N. (2008). “Fungal viruses,” in Encyclopedia of Virology. eds. B. W. J. Mahy and M. H. V. Van Regenmortel, 3rd Edn. Elsevier, 284–291.
Gonzalez, M. F., Magdama, F., Galarza, L., Sosa, D., and Romero, C. (2020). Evaluation of the sensitivity and synergistic effect of Trichoderma reesei and mancozeb to inhibit under in vitro conditions the growth of fusarium oxysporum. Commun. Integr. Biol. 13, 160–169. doi: 10.1080/19420889.2020.1829267
Grabherr, M. G., Haas, B. J., Yassour, M., Levin, J. Z., Thompson, D. A., Amit, I., et al. (2011). Trinity: reconstructing a full-length transcriptome without a genome from RNA-Seq data. Nat. Biotechnol. 29, 644–652. doi: 10.1038/nbt.1883
Hanada, R. E., Pomella, A. W. V., Costa, H. S., Bezerra, J. L., Loguercio, L. L., and Pereira, J. O. (2010). Endophytic fungal diversity in Theobroma cacao (cacao) and T. grandiflorum (cupuaçu) trees and their potential for growth promotion and biocontrol of black-pod disease. Fungal Biol. 114, 901–910. doi: 10.1016/j.funbio.2010.08.006
Harman, G. E. (2006). Overview of mechanisms and uses of Trichoderma spp. Phytopathology 96, 190–194. doi: 10.1094/PHYTO-96-0190
Hidalgo, E., Bateman, R., Krauss, U., ten Hoopen, M., and Martínez, A. (2003). A field investigation into delivery systems for agents to control Moniliophthora roreri. Eur. J. Plant Pathol. 109, 953–961. doi: 10.1023/B:EJPP.0000003746.16934.e2
Hillman, B. I., and Cai, G. (2013). The family narnaviridae: simplest of RNA viruses. Adv. Virus Res. 86, 149–176. doi: 10.1016/B978-0-12-394315-6.00006-4
Hipólito-Romero, E., Cocoletzi-Vásquez, E., Ramos-Prado, J. M., Espinoza, C., Torres-de la Cruz, M., and Ricaño-Rodríguez, J. (2020). Breve aproximación a la naturaleza genómica de Moniliophthora roreri CPMRT01 aislado de cacao en Tabasco, México//brief approach to the genomic nature of Moniliophthora roreri CPMRT01 isolated from cocoa in Tabasco, Mexico. Biotecnia 22, 39–49. doi: 10.18633/biotecnia.v22i2.1244
Hu, Y., and Zhu, B. (2016). Study on genetic engineering of acremonium chrysogenum, the cephalosporin C producer. Synth. Syst. Biotechnol. 1, 143–149. doi: 10.1016/j.synbio.2016.09.002
Huang, X., and Madan, A. (1999). CAP3: a DNA sequence assembly program. Genome Res. 9, 868–877. doi: 10.1101/gr.9.9.868
Jiang, L., Jeong, J. C., Lee, J.-S., Park, J. M., Yang, J.-W., Lee, M. H., et al. (2019). Potential of Pantoea dispersa as an effective biocontrol agent for black rot in sweet potato. Sci. Rep. 9:16354. doi: 10.1038/s41598-019-52804-3
Johnson, E., Rutherford, M. A., Edgington, S., Flood, J., Crozier, J., Cafá, G., et al. (2017). First report of Moniliophthora roreri causing frosty pod rot on Theobroma cacao in Jamaica. New Dis. Rep. 36:2. doi: 10.5197/j.2044-0588.2017.036.002
Katoh, K., Rozewicki, J., and Yamada, K. D. (2019). MAFFT online service: multiple sequence alignment, interactive sequence choice and visualization. Brief. Bioinform. 20, 1160–1166. doi: 10.1093/bib/bbx108
Katz, D. L., Doughty, K., and Ali, A. (2011). Cocoa and chocolate in human health and disease. Antioxid. Redox Signal. 15, 2779–2811. doi: 10.1089/ars.2010.3697
Krauss, U., Martijn ten Hoopen, G., Hidalgo, E., Martínez, A., Stirrup, T., Arroyo, C., et al. (2006). The effect of cane molasses amendment on biocontrol of frosty pod rot (Moniliophthora roreri) and black pod (Phytophthora spp.) of cocoa (Theobroma cacao) in Panama. Biol. Control 39, 232–239. doi: 10.1016/j.biocontrol.2006.06.005
Langmead, B., and Salzberg, S. L. (2012). Fast gapped-read alignment with bowtie 2. Nat. Methods 9, 357–359. doi: 10.1038/nmeth.1923
Lefkowitz, E. J., Dempsey, D. M., Hendrickson, R. C., Orton, R. J., Siddell, S. G., and Smith, D. B. (2018). Virus taxonomy: the database of the international committee on taxonomy of viruses (ICTV). Nucleic Acids Res. 46, D708–D717. doi: 10.1093/nar/gkx932
Leiva, S., Oliva, M., Hernández, E., Chuquibala, B., Rubio, K., García, F., et al. (2020). Assessment of the potential of Trichoderma spp. strains native to Bagua (Amazonas, Peru) in the biocontrol of frosty pod rot (Moniliophthora roreri). Agronomy 10:1376. doi: 10.3390/agronomy10091376
Li, Y., Zhou, M., Yang, Y., Liu, Q., Zhang, Z., Han, C., et al. (2021). Characterization of the Mycovirome from the plant-pathogenic fungus Cercospora beticola. Viruses 13:1915. doi: 10.3390/v13101915
Lin, Y., Zhou, J., Zhou, X., Shuai, S., Zhou, R., An, H., et al. (2020). A novel narnavirus from the plant-pathogenic fungus Magnaporthe oryzae. Arch. Virol. 165, 1235–1240. doi: 10.1007/s00705-020-04586-7
Liu, Y., Zhang, L., Esmael, A., Duan, J., Bian, X., Jia, J., et al. (2020). Four novel botourmiaviruses co-infecting an isolate of the rice blast fungus Magnaporthe oryzae. Viruses 12:E1383. doi: 10.3390/v12121383
Marelli, J.-P., Guest, D. I., Bailey, B. A., Evans, H. C., Brown, J. K., Junaid, M., et al. (2019). Chocolate under threat from old and new cacao diseases. Phytopathology 109, 1331–1343. doi: 10.1094/PHYTO-12-18-0477-RVW
Marelli, J.-P., Maximova, S. N., Gramacho, K. P., Kang, S., and Guiltinan, M. J. (2009). Infection biology of Moniliophthora perniciosa on Theobroma cacao and alternate solanaceous hosts. Trop. Plant Biol. 2, 149–160. doi: 10.1007/s12042-009-9038-1
Maximova, S. N., Marelli, J.-P., Young, A., Pishak, S., Verica, J. A., and Guiltinan, M. J. (2006). Over-expression of a cacao class I Chitinase gene in Theobroma cacao L. enhances resistance against the pathogen, Colletotrichum gloeosporioides. Planta 224, 740–749. doi: 10.1007/s00425-005-0188-6
McElroy, M. S., Navarro, A. J. R., Mustiga, G., Stack, C., Gezan, S., Peña, G., et al. (2018). Prediction of cacao (Theobroma cacao) resistance to Moniliophthora spp. diseases via genome-wide association analysis and genomic selection. Front. Plant Sci. 9:343. doi: 10.3389/fpls.2018.00343
McSpadden Gardener, B. B. (2007). Diversity and ecology of biocontrol Pseudomonas spp. in agricultural systems. Phytopathology 97, 221–226. doi: 10.1094/PHYTO-97-2-0221
Meinhardt, L. W., Costa, G. G. L., Thomazella, D. P. T., Teixeira, P. J. P. L., Carazzolle, M. F., Schuster, S. C., et al. (2014). Genome and secretome analysis of the hemibiotrophic fungal pathogen, Moniliophthora roreri, which causes frosty pod rot disease of cacao: mechanisms of the biotrophic and necrotrophic phases. BMC Genomics 15:164. doi: 10.1186/1471-2164-15-164
Meinhardt, L. W., Rincones, J., Bailey, B. A., Aime, M. C., Griffith, G. W., Zhang, D., et al. (2008). Moniliophthora perniciosa, the causal agent of witches’ broom disease of cacao: what’s new from this old foe? Mol. Plant Pathol. 9, 577–588. doi: 10.1111/j.1364-3703.2008.00496.x
Mora-Ocampo, I. Y., Pirovani, C. P., Luz, E. D. M. N., Rêgo, A. P. B., Silva, E. M. A., Rhodes-Valbuena, M., et al. (2021). Ceratocystis cacaofunesta differentially modulates the proteome in xylem-enriched tissue of cocoa genotypes with contrasting resistance to Ceratocystis wilt. Planta 254:94. doi: 10.1007/s00425-021-03747-5
Motamayor, J. C., Risterucci, A. M., Lopez, P. A., Ortiz, C. F., Moreno, A., and Lanaud, C. (2002). Cacao domestication I: the origin of the cacao cultivated by the Mayas. Heredity 89, 380–386. doi: 10.1038/sj.hdy.6800156
Nascimento, A. D., Lima, M. O., Feijó, F. M., Júnior, J. H., Sobrinho, R. R., Assunção, I. P., et al. (2019). First report of Colletotrichum aeschynomenes causing anthracnose in cacao (Theobroma cacao) in Brazil. Plant Dis. 103:3284. doi: 10.1094/PDIS-11-18-2047-PDN
Nguyen, L.-T., Schmidt, H. A., von Haeseler, A., and Minh, B. Q. (2015). IQ-TREE: a fast and effective stochastic algorithm for estimating maximum-likelihood phylogenies. Mol. Biol. Evol. 32, 268–274. doi: 10.1093/molbev/msu300
Osorio-Guarín, J. A., Berdugo-Cely, J. A., Coronado-Silva, R. A., Baez, E., Jaimes, Y., and Yockteng, R. (2020). Genome-wide association study reveals novel candidate genes associated with productivity and disease resistance to Moniliophthora spp. in cacao (Theobroma cacao L.). G3 10, 1713–1725. doi: 10.1534/g3.120.401153
Pachoute, J., Nascimento, V. L., and de Souza, D. J. (2021). Beauveria bassiana enhances the growth of cowpea plants and increases the mortality of Cerotoma arcuata. Curr. Microbiol. 78, 3762–3769. doi: 10.1007/s00284-021-02638-y
Pakora, G.-A., Mpika, J., Kone, D., Ducamp, M., Kebe, I., Nay, B., et al. (2018). Inhibition of phytophthora species, agents of cocoa black pod disease, by secondary metabolites of Trichoderma species. Environ. Sci. Pollut. Res. Int. 25, 29901–29909. doi: 10.1007/s11356-017-0283-9
Peix, A., Ramírez-Bahena, M.-H., and Velázquez, E. (2009). Historical evolution and current status of the taxonomy of genus pseudomonas. Infect. Genet. Evol. 9, 1132–1147. doi: 10.1016/j.meegid.2009.08.001
Phillips-Mora, W., Aime, M., and Wilkinson, M. (2007). Biodiversity and biogeography of the cacao (Theobroma cacao) pathogen Moniliophthora roreri in tropical America. Plant Pathol. 56, 911–922. doi: 10.1111/j.1365-3059.2007.01646.x
Priya, P., Aneesh, B., and Harikrishnan, K. (2021). Genomics as a potential tool to unravel the rhizosphere microbiome interactions on plant health. J. Microbiol. Methods 185:106215. doi: 10.1016/j.mimet.2021.106215
R Core Team (2021). R: A Language and Environment for Statistical Computing. Version:4.1.2. Vienna: R Foundation for Statistical Computing.
Reyes-Figueroa, O., Ortiz-García, C. F., Torres-de la Cruz, M., del Lagunes-Espinoza, L. C., Valdovinos-Ponce, G., Reyes-Figueroa, O., et al. (2016). Trichoderma species from the cacao agroecosystem with biocontrol potential of Moniliophthora roreri. Revista Chapingo Serie Ciencias Forestales y Del Ambiente XXII, 149–163. doi: 10.5154/r.rchscfa.2015.08.036
Rojas, E. I., Rehner, S. A., Samuels, G. J., Van Bael, S. A., Herre, E. A., Cannon, P., et al. (2010). Colletotrichum gloeosporioides s.l. associated with Theobroma cacao and other plants in Panama: multilocus phylogenies distinguish host-associated pathogens from asymptomatic endophytes. Mycologia 102, 1318–1338. doi: 10.3852/09-244
Rubini, M. R., Silva-Ribeiro, R. T., Pomella, A. W. V., Maki, C. S., Araújo, W. L., dos Santos, D. R., et al. (2005). Diversity of endophytic fungal community of cacao (Theobroma cacao L.) and biological control of Crinipellis perniciosa, causal agent of witches’ broom disease. Int. J. Biol. Sci. 1, 24–33. doi: 10.7150/ijbs.1.24
Sahin, E., and Akata, I. (2019). Complete genome sequence of a novel mitovirus from the ectomycorrhizal fungus Geopora sumneriana. Arch. Virol. 164, 2853–2857. doi: 10.1007/s00705-019-04367-x
Saminathan, T., García, M., Ghimire, B., Lopez, C., Bodunrin, A., Nimmakayala, P., et al. (2018). Metagenomic and Metatranscriptomic analyses of diverse watermelon cultivars reveal the role of fruit associated microbiome in carbohydrate metabolism and ripening of mature fruits. Front. Plant Sci. 9:4. doi: 10.3389/fpls.2018.00004
Samuels, G. J., Suarez, C., Solis, K., Holmes, K. A., Thomas, S. E., Ismaiel, A., et al. (2006). Trichoderma theobromicola and T. paucisporum: two new species isolated from cacao in South America. Mycol. Res. 110, 381–392. doi: 10.1016/j.mycres.2006.01.009
Sánchez, F., Quevedo, U. T. E.de, Garcés, F., and Quevedo, U. T. E.de. (2012). Moniliophthora roreri (Cif y par) Evans et al. In the crop of cocoa. Universidad Nacional de Trujillo. Available at: https://scholar.google.com/scholar_lookup?title=Moniliophthora+roreri+%28Cif+y+Par%29+Evans+et+al.+in+the+crop+of+cocoa&author=S%C3%A1nchez%2C+Fernando%3B+Universidad+T%C3%A9cnica+Estatal+de+Quevedo&publication_year=2012 (Accessed September 22, 2022).
Sánchez-Fernández, R. E., Lorena Sánchez-Ortiz, B., Monserrat Sandoval-Espinosa, Y. K., Ulloa-Benítez, Á., Armendáriz-Guillén, B., Claudia García-Méndez, M., et al. (2013). Hongos endófitos: Fuente potencial de metabolitos secundarios bioactivos con utilidad en agricultura y medicina. Tip Rev. Espec. Cienc. Quím.-Biol. 16, 132–146. doi: 10.1016/S1405-888X(13)72084-9
Schoch, C. L., Ciufo, S., Domrachev, M., Hotton, C. L., Kannan, S., Khovanskaya, R., et al. (2020). NCBI taxonomy: a comprehensive update on curation, resources and tools. J. Biol. Databases Curation 2020:baaa062. doi: 10.1093/database/baaa062
Serra, J. L., Moura, F. G., de Pereira, G. V. M., Soccol, C. R., Rogez, H., and Darnet, S. (2019). Determination of the microbial community in Amazonian cocoa bean fermentation by Illumina-based metagenomic sequencing. LWT 106, 229–239. doi: 10.1016/j.lwt.2019.02.038
Shahanas, E., Panjikkaran, S. T., Aneena, E. R., Sharon, C. L., and Remya, P. R. (2019). Health benefits of bioactive compounds from cocoa (Theobroma cacao). Agric. Rev. 40, 143–149. doi: 10.18805/ag.R-1851
Simamora, A. V., Hahuly, M. V., and Henuk, J. B. (2021). Endophytic fungi as potential biocontrol agents of Phytophthora palmivora in the cocoa plant. Biodiversitas 22:5. doi: 10.13057/biodiv/d220519
Son, M., Yu, J., and Kim, K.-H. (2015). Five Questions about Mycoviruses. PLoS Pathog. 11:e1005172. doi: 10.1371/journal.ppat.1005172
Suárez, L. Y., and Cabrales, C. P. (2008). Identificación de especies de cepas nativas de Trichoderma sp. Y Bacillus sp. Y evaluación de su potencial antagonista in vitro frente al hongo fitopatógeno nativo Moniliophthora roreri en el departamento de Norte de Santander. Respuestas 13, 45–56.
Sutela, S., Forgia, M., Vainio, E. J., Chiapello, M., Daghino, S., Vallino, M., et al. (2020). The virome from a collection of endomycorrhizal fungi reveals new viral taxa with unprecedented genome organization. Virus Evol. 6:veaa076. doi: 10.1093/ve/veaa076
Tamchek, N., Lee, P.-C., and Rosmin, K. (2019). Metatranscriptomic sequencing and analysis of cacao rhizosphere. 11, 108–115.
Tchinda, R. A. M., Boudjeko, T., Simao-Beaunoir, A.-M., Lerat, S., Tsala, É., Monga, E., et al. (2016). Morphological, physiological, and taxonomic characterization of actinobacterial isolates living as endophytes of cacao pods and cacao seeds. Microbes Environ. 31, 56–62. doi: 10.1264/jsme2.ME15146
Teixeira, P. J. P. L., de Thomazella, D. P. T., and Pereira, G. A. G. (2015). Time for chocolate: current understanding and new perspectives on cacao witches’ broom disease research. PLoS Pathog. 11:e1005130. doi: 10.1371/journal.ppat.1005130
Teixeira, P. J. P. L., de Thomazella, D. P. T., Reis, O., do Prado, P. F. V., do Rio, M. C. S., Fiorin, G. L., et al. (2014). High-resolution transcript profiling of the atypical biotrophic interaction between Theobroma cacao and the fungal pathogen Moniliophthora perniciosa. Plant Cell 26, 4245–4269. doi: 10.1105/tpc.114.130807
Urich, T., Lanzén, A., Qi, J., Huson, D. H., Schleper, C., and Schuster, S. C. (2008). Simultaneous assessment of soil microbial community structure and function through analysis of the meta-transcriptome. PLoS One 3:e2527. doi: 10.1371/journal.pone.0002527
Verce, M., Schoonejans, J., Hernandez Aguirre, C., Molina-Bravo, R., De Vuyst, L., and Weckx, S. (2021). A combined metagenomics and metatranscriptomics approach to unravel Costa Rican cocoa box fermentation processes reveals yet unreported microbial species and functionalities. Front. Microbiol. 12:245. doi: 10.3389/fmicb.2021.641185
Keywords: Theobroma cacao, microbiota, metatranscriptomics, frosty pod rot disease, virus, narnavirus, fungi
Citation: Reyes BMD, Fonseca PLC, Heming NM, Conceição LBdA, Nascimento KTS, Gramacho KP, Arevalo-Gardini E, Pirovani CP and Aguiar ERGR (2023) Characterization of the microbiota dynamics associated with Moniliophthora roreri, causal agent of cocoa frosty pod rot disease, reveals new viral species. Front. Microbiol. 13:1053562. doi: 10.3389/fmicb.2022.1053562
Edited by:
Yong Wang, Guizhou University, ChinaReviewed by:
Zi Shi, Beijing Academy of Agriculture and Forestry Sciences, ChinaChirlei Glienke, Federal University of Paraná, Brazil
Copyright © 2023 Reyes, Fonseca, Heming, Conceição, Nascimento, Gramacho, Arevalo-Gardini, Pirovani and Aguiar. This is an open-access article distributed under the terms of the Creative Commons Attribution License (CC BY). The use, distribution or reproduction in other forums is permitted, provided the original author(s) and the copyright owner(s) are credited and that the original publication in this journal is cited, in accordance with accepted academic practice. No use, distribution or reproduction is permitted which does not comply with these terms.
*Correspondence: Eric Roberto Guimarães Rocha Aguiar, ✉ ericgdp@gmail.com