- 1Zhejiang Province Key Laboratory of Plant Secondary Metabolism and Regulation, College of Life Sciences and Medicine, Zhejiang Sci-Tech University, Hangzhou, China
- 2Key Laboratory of Conservation Biology for Endangered Wildlife of the Ministry of Education, Laboratory of Systematic & Evolutionary Botany and Biodiversity, College of Life Sciences, Zhejiang University, Hangzhou, China
- 3Key Laboratory of Biological Resources and Conservation and Application, College of Life Sciences, Tarim University, Alaer, China
- 4Department of Biosciences, Salzburg University, Salzburg, Austria
Numerous temperate plants and animals on the Qinghai-Tibet Plateau (QTP) are hypothesized to have differentiated due to vicariant allopatric speciation associated with the geologic uplifts. However, this hypothesis has rarely been tested through a phylogeographic study of relative species in a broader geographic context, including the QTP, Tianshan Mountains, Mongolian Plateau, and surrounding regions. To understand the speciation and diversification process of plants across this wide area, phylogeographic analysis were examined from Scrophularia incisa and two other closely relative species comprising S. kiriloviana and S. dentata. Thirty-two populations of the three close relatives were genotyped using chloroplast DNA fragments and nuclear microsatellite loci to assess population structure and diversity, supplemented by phylogenetic dating, ancestral area reconstructions and species distribution modelings, as well as niche identity tests. Our chloroplast DNA (cpDNA) phylogeny showed that this monophyletic group of desert and steppe semi-shrub is derived from a Middle Pliocene ancestor of the Central Asia. Lineages in Central Asia vs. China diverged through climate/tectonic-induced vicariance during Middle Pliocene. Genetic and ENM data in conjunction with niche differentiation analyses support that the divergence of S. incisa, S. dentata and S. kiriloviana in China lineage proceeded through allopatric speciation, might triggered by early Pleistocene climate change of increase of aridification and enlargement of deserts, while subsequent climate-induced cycles of range contractions/expansions enhanced the geographical isolation and habit fragmentation of these taxa. These findings highlight the importance of the Plio-Pleistocene climate change in shaping genetic diversity and driving speciation in temperate steppes and deserts of Northwestern China.
Introduction
Phylogeography is a field of study concerned with principles and processes governing the geographic distributions of genealogical lineages, especially those within and among closely related species (Avise, 2000). A core objective of phylogeographic study is to recognize the responses of organisms to the past climatic oscillations, population biology and evolutionary scenarios within and between species (Abbott et al., 2000; Avise, 2009; Liu et al., 2012). In China, previous plant phylogeographic studies have broadly focused on three floristic regions or “subkingdoms” (sensu lato; Wu and Wu, 1996), including the Qinghai-Tibet Plateau (QTP), the “Sino-Himalayan”/Hengduan Mountain region of Southwest China, and the “Sino-Japanese” region of subtropical (Central-South-East) China and areas further north of the Yangtze River (Qiu et al., 2011; Liu et al., 2012). Together, these regions harbor the largest amount of temperate plant species diversity in the world (Myers et al., 2000; Wen et al., 2014). The arid Northwestern China is located in the interior of Eurasia at a profound distance from oceans and consequently reached by little moisture, with early uplift of the QTP, westerly winds weakened and the Mongolian-Siberian high pressure intensified, resulting in less precipitation and greater cold in this region (Fang et al., 2002a; Guo et al., 2002; Wen et al., 2016). Increased aridity with continued uplift of mountain ranges expedited the process of desertification in Northwestern China and caused large-scale expansion of deserts, such as the formation of Gurbantunggut Desert of the Junggar Basin and the Taklimakan Desert of the Tarim Basin (Sun et al., 1998; Fang et al., 2002a,b; Ding et al., 2005; Shi et al., 2005), which might have acted as an effective promoter to further adaptation of plants to various desert habitats (Wang et al., 2013; Wen et al., 2016). Even though aridification and desert formation occurred in the interior of Eurasia since the India-Asia continental collision (<ca. 50 million years age, Mya), the consequent extreme aridity and expansion of deserts in Northwest China is probably of early Miocene origin (ca. 23–16 Mya) and related to extensive uplifts of the QTP (Miao et al., 2011; Hoorn et al., 2012; Zhang et al., 2012). Moreover, some phylogeographic studies have specifically dealt with a single species of the arid flora of Northwest China, put more emphasis on the role of Quaternary climate oscillations in shaping geographical patterns of intraspecific genetic diversity and triggering glacial contractions and inter-/postglacial expansions (Li et al., 2012; Ma et al., 2012; Meng et al., 2015). However, phylogeographic study about understanding the speciation and diversification process of closely related plant taxa in this broad arid region (including the QTP, Tianshan Mountains, Mongolian Plateau, and surrounding regions) is rarely few.
The genus Scrophularia (Scrophulariaceae) comprises more than 200 species of mainly Holartic distribution in both the Old and New World (Willis, 1973; Hong, 1983; Mabberley, 1997; Hong et al., 1998). The two sections recognized (Stiefelhagen, 1910) are: sect. Scrophualria characterized by perennial herbs and sect. Caninae characterized by perennial semi-shrubs and xerophytes, as mainly distributed in Northwest China and Central Asia (Chen et al., 2014a; Wang et al., 2018). Here, we report a phylogeographic study of the “Scrophularia incisa complex” (sect. Caninae), comprising three closely related species that are mainly (but not exclusively) distributed in Northwest China and also used for medicinal purposes of treatment of exanthema and fever in Traditional Tibetan Medicine (TTM) and Mogolian Medicine (TMM), due to its secondary metabolites of the high ingredient of iridoids and phenylpropanoids (Zhang et al., 2013; Yang et al., 2014; Zhang L. Q. et al., 2014). Our preliminary analyses of nuclear ribosomal (nr) and chloroplast (cp) DNA placed this species complex as a monophyletic group embedded in sect. Caninae clade (Wang, 2015). Of those, S. incisa Weinm. presents a belt-like distribution mainly in Qinghai and Gansu Provinces as well as in Inner Mongolia of northern China, stretching westward to Central Asia and eastward to Siberia, Russia (Hong et al., 1998; Wang et al., 2014); S. dentata Royle ex Benth. occurs in the western and southern Tibet, northwestern India and Pakistan; and S. kiriloviana Schischk. is found in Xinjiang Province and Central Asia (Hong et al., 1998). All three species have very similar habitat preferences (e.g., floodplains, grasslands, and mountain valleys) and mainly differ in leaf shape and aspects of the calyx membrane (Figure 1). In detail, the leaves of S. incisa are toothed to lobed, rarely basally 1- or 2- segmented, with narrowly membranous margin on calyx lobes at anthesis; the leaves of S. dentata are lobed, deeply serrated, while the calyx lobes at anthesis have no conspicuous membranous margin, but which is obvious at the time of fruiting. Finally, in S. kiriloviana, the degree of leaf lobed is often apically toothed or coarsely serrate to pinnately parted, basally pinnately parted to pinnatisect, this species has a broadly membranous calyx lobe margin (Hong et al., 1998). Given the widespread distribution of the complex species (from Central Asia to Far East), we focused our phylogeographic study to medicinal species populations in Northwestern China to investigate their patterns of genetic diversity, structure and differentiation, with the overall aim to elucidate their spatiotemporal patterns of divergence and underlying causes.
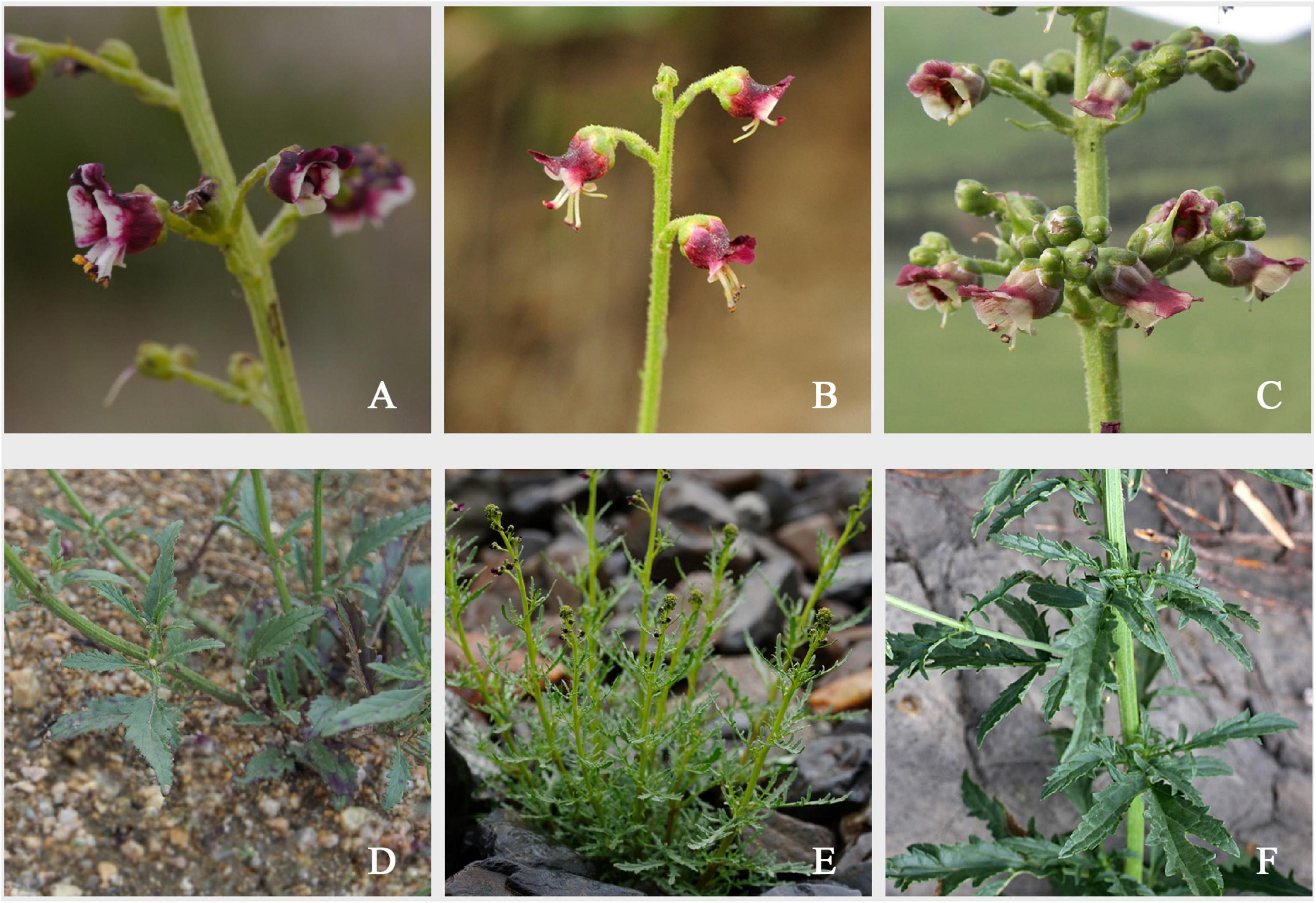
Figure 1. Flower and leaves morphology of the three studied species. (A) Flower of S. incisa. (B) Flower of S. dentata. (C) Flower of S. kiriloviana. (D) Leaves of S. incisa. (E) Leaves of S. dentata. (F) Leaves of S. kiriloviana. Photo A by Ye-Chun Xu; B by Jun Liu; C, F by Xue-Jing Zhan; D, E by Guo-Jun Hua.
Mechanisms of species diversification on the QTP and adjacent regions have attracted the attention for many years, and more than one mechanism may have played a role for a certain plant group (Qiu et al., 2011; Wen et al., 2014). Reviewing recent evidences from phylogenetic and biogeographic studies in plants, vicariant allopatric speciation associated with the geologic uplifts has been proposed as the main mechanism of species diversification on the QTP and adjacent regions (Yang et al., 2009; Xu et al., 2010). The second pattern indicates that the Tertiary and Quaternary climatic oscillations associated with the QTP uplifts have triggered and facilitated speciation and diversification, and shaped geographic genetic structure and the recolonization patterns from multiple refugia (Hewitt, 2000, 2004; Cun and Wang, 2010; Liu et al., 2012). The third pattern suggests that hybridization and introgression have contributed to the high species diversity on the QTP and adjacent areas, because of secondary sympatry during relatively stable stages between different uplifts of the QTP (Liu et al., 2006; Wang et al., 2010; Wen et al., 2014). Moreover, morphological convergence and innovations (Wang et al., 2009; Xie et al., 2011; Zhang J. Q. et al., 2014), biotic interactions (pollinator-mediated isolation, Hou et al., 2008; Niu et al., 2011; Eaton et al., 2012), and polyploidy (Stebbins, 1940, 1971; Mayrose et al., 2011) are also considered to be the important mechanisms in plant evolution.
In the present study, we used maternally inherited chloroplast DNA (cpDNA) and bi-parentally inherited nuclear microsatellite (nSSR) data, combing the (palaeo-)climatic data and distribution models, in an attempt to investigate the phylogeography and spatial-temporal process of divergence within the S. incisa complex. The specific aims of this study were: (1) to illuminate the phylogeographic pattern of the species complex based on plastid vs. nuclear data and species boundaries; (2) to determine the divergence times of the three focal species and intraspecific lineages as well as the underlying divergence mechanism; (3) to infer the influence of Quaternary climatic oscillations on the species complex.
Materials and methods
Plant materials and sampling design
In total, 522 individuals of the S. incisa complex were collected, including 263 individuals of S. incisa (11 populations from in Qinghai Province, 3 from Gansu Province, 1 from Northeastern Inner Mongolia), 232 individuals of S. kiriloviana (15 populations mainly distributed in Xinjiang Province), and 27 individuals of S. dentata (three populations from Tibet). According to our field investigations, the current population number of S. incisa in the Central and Western regions of Inner Mongolia is limited, possibly as a consequence of habitat loss due to over-exploitation and drought. Our sampling covers most of the distribution range of this species complex. All samples (n = 522) were surveyed for nSSR variation (except for the CA population of S. kiriloviana from West Tien-Shan in Central Asia because of its small sample size); for cpDNA, all samples of S. dentata (n = 27) were sequenced, but only subsets for S. incisa (n = 202) and S. kiriloviana (n = 177).
DNA extraction, sequencing, and microsatellite genotyping
Total genomic DNA was extracted from leaf material that had been dried with silica-gel, using DNA Plantzol (Invitrogen, Carlsbad, CA, USA) following the manufacturer’s protocol. For the phylogeographic DNA surveys, we sequenced two intergenic spacer (IGS) regions of cpDNA (trnL-trnF and psbA-trnH). To better resolve the phylogenetic relationships among the 30 haplotypes recovered from the above two cpDNA markers, one additional cpDNA region (trnQ-rps16) was sequenced for 30 samples representative of all the haplotypes identified in the phylogeographic survey. PCR was performed using the primer sequences and amplification conditions of Scheunert and Heubl (2011, 2014, 2017). Sequences were generated with an ABI 377XL DNA-sequencer, and edited, assembled, and aligned in GENEIOUS PRO v4.8.2 (Drummond et al., 2009; available at http://www.geneious.com). All haplotype sequences identified in the present study were deposited in GenBank (see Supplementary Table 2 for accession numbers).
All DNA samples (except for population CA) were genotyped at 12 nSSR loci using primers (Scin1–12; GenBank accession numbers: JQ773338–773349) and amplification protocols developed for S. incisa (Wang et al., 2014; see Supplementary Table 3). PCR products were separated on a MegaBACE 1000 (CE Healthcare Biosciences, Sunnyvale, California, USA). Alleles were scored manually using GENETIC PROFILER v2.2 (GE Healthcare Biosciences).
Phylogeographical and population genetic analyses of chloroplast DNA and nSSRs
For cpDNA, haplotypes (h) and nucleotide (π) diversities were estimated using DNASP v 5.1 (Librado and Rozas, 2009) at the levels of populations (hS, πS) and species (hT, πT). Networks indicating the genealogical relationships of the haplotypes were constructed in TCS v 1.21 (Clement et al., 2000) under the 95% statistical parsimony criterion. For cpDNA, we had to increase the TCS connection limit to 50 steps to link the divergent networks of the three species. Gaps (indels) detected in the cpDNA dataset were treated as single mutation events, and coded as substitutions (A or T).
The program PERMUT (Pons and Petit, 1996) was employed to compare parameters of cpDNA-based population differentiation (NST and GST) of each species and/or lineage separately based on 1,000 random permutations. A mismatch distribution analysis (MDA; Slatkin and Maddison, 1989; Rogers and Harpending, 1992; Schneider and Excoffier, 1999) was conducted to examine the demographic expansions of the four major cpDNA clades identified in the phylogenetic analyses. As population structure has a limited effect on the mismatch distribution (Rogers, 1995; Bernatchez, 2001), we pooled all haplotypes of each clade and did not consider their frequencies. We used 1,000 parametric bootstrap replicates to generate an expected distribution using a model of sudden demographic expansion (Excoffier and Lischer, 2010), to calculate the sum of squared deviations (SSD) and raggedness index (HRag) of Harpending (1994) between observed and expected mismatch distributions and to obtain 95% confidence intervals (CIs) around τ. We also calculated Tajima’s (1989) D and Fu’s (1997) Fs to assess possible expansions. The D and Fs statistics should have large negative values within a clade under the expansion hypothesis due to an excess of rare new mutations. We calculated significance of the tests with 10,000 replicates. All of these demographic tests were performed using ARLEQUIN v.3.5 (Excoffier and Lischer, 2010). When sudden expansions were detected, we used the equation τ = 2ut (Rogers and Harpending, 1992; Rogers, 1995) to estimate expansion times, where t is the expansion time in number of generations, τ is the mode of the mismatch distribution, and u is the mutation rate per generation for the whole analyzed sequence. We calculated u according to u = μkg, where μ is the substitution rate per nucleotide site per year of the combined cpDNA regions obtained from the corresponding clock-calibrated BEAST tree (see below), k is the average sequence length of the DNA region under study (here, 1,310 bp), and g is the generation time in years (i.e., age of first reproduction); for, g, we assumed 3 years, according to our observation on S. incisa (R.H. Wang, pers.obs.).
For the nSSRs, we calculated measures of genetic diversity for each population, and across all 12 loci. The following diversity and inbreeding parameters were calculated: AR, allelic richness (Mousadik and Petit, 1996); PAR, private allelic richness; HE, expected heterozygosity (Nei, 1978), HO, observed heterozygosity; and the average inbreeding coefficient (FIS) across all loci. AR and PAR were calculated by rarifying to 12 gene copies using FSTAT and HP-RARE v1.1 (Kalinowski, 2005), respectively.
Genetic subgroups in the nSSR dataset were identified by a Bayesian analysis in STRUCTURE v2.3 (Pritchard et al., 2009) using the admixture model and assuming independent allele frequencies among populations. The number of clusters (K) was set to vary from 1 to 10. For each value of K, we performed 10 runs with a burn-in length of 10,000 and a run length of 100,000 Markov chain Monte Carlo (MCMC) replications. Two alternative methods were used to explore the true number of gene pools: by monitoring the change in average of log-likelihood of the data, logeP(D), of independent runs for each K, following Pritchard et al. (2000), and by observing the second-order rate of change of logeP(D) between successive K values (Evanno et al., 2005).
In order to quantify variation in cpDNA sequences and nSSRs among populations and clades (as identified by the TCS network and BEAST-derived tree; see below), non-hierarchical and hierarchical analyses of molecular variance (AMOVAs) were carried out in ARLEQUIN, using Φ- and R-statistics, respectively. The significance of fixation indices was tested using 10,000 permutations.
Gene flow analyses
In order to test whether postglacial gene flow may obscure the genetic signatures of historical population processes, based on the nSSR dataset, we obtained pairwise estimates of postglacial gene flow (c. 4Ne generations in the past; Beerli and Felsenstein, 2001) between regional population groups (i.e., four genealogically distinct units of cpDNA) using model-based coalescent analysis in MIGRATE v3.1.3 (Beerli, 2006). This program calculates maximum likelihood (ML) estimates for mutation-scaled migration rate (M) [M = mμ–1, where μ is the mutation rate per generation (3 × 10–3); Udupa and Baum, 2001]. These analyses were run for three replicates under a Brownian motion mutation model with constant mutation rates for all loci and starting parameters based on FST calculations. We used uniform priors and Metropolis sampling with 10 short and five long chains with 10,000 and 100,000 sampled genealogies, respectively. Genealogies were 50 steps apart and the first 10,000 were discarded as burn-in. We used a static heating scheme at four temperatures (1, 1.5, 3, and 6) to efficiently search the genealogy space.
Phylogenetic divergence time estimation
Divergence time between cpDNA haplotype lineages was estimated under ML and Bayesian inference (BI) approaches, with six outgroup species, including S. integrifolia of sect. Caninae (a close relative according to Scheunert and Heubl, 2017), three species of sect. Scrophularia (S. henryi, S. takesimensis, and S. buergeriana), and two species of Verbascum (V. chinense and V. phoeniceum). Taking advantage of calibration points used in a previous phylogenetic study of Lamiales (Xu et al., 2018), we adopted the estimated median crown age of Scrophularia [mean, 7.88 Ma; 95% highest posterior density (HPD) interval, 2.67–14.75 Ma] to calibrate the respective root node in our tree (node 1 in Figure 2). A Yule process was specified as a tree prior. Bayesian searches were conducted in BEAST (Drummond and Rambaut, 2007) using a GTR + I + G substitution model selected by JMODELTEST (Posada, 2008) and an uncorrelated lognormal relaxed clock (Drummond et al., 2002). For the BEAST analysis, MCMC runs were performed, each of 5 × 107 generations, following a burn-in of the initial 10% cycles. MCMC samples were inspected in TRACER to confirm sampling adequacy and convergence of the chains to a stationary distribution. In addition, haplotype relationships were also inferred via ML analysis in RAXML v7.2.8 (Stamatakis et al., 2008) under the GTR + G substitution model (as selected by JMODELTEST), and with gaps (indels) treated as missing data. Node support was assessed using 1,000 “fast bootstrap” replicates.
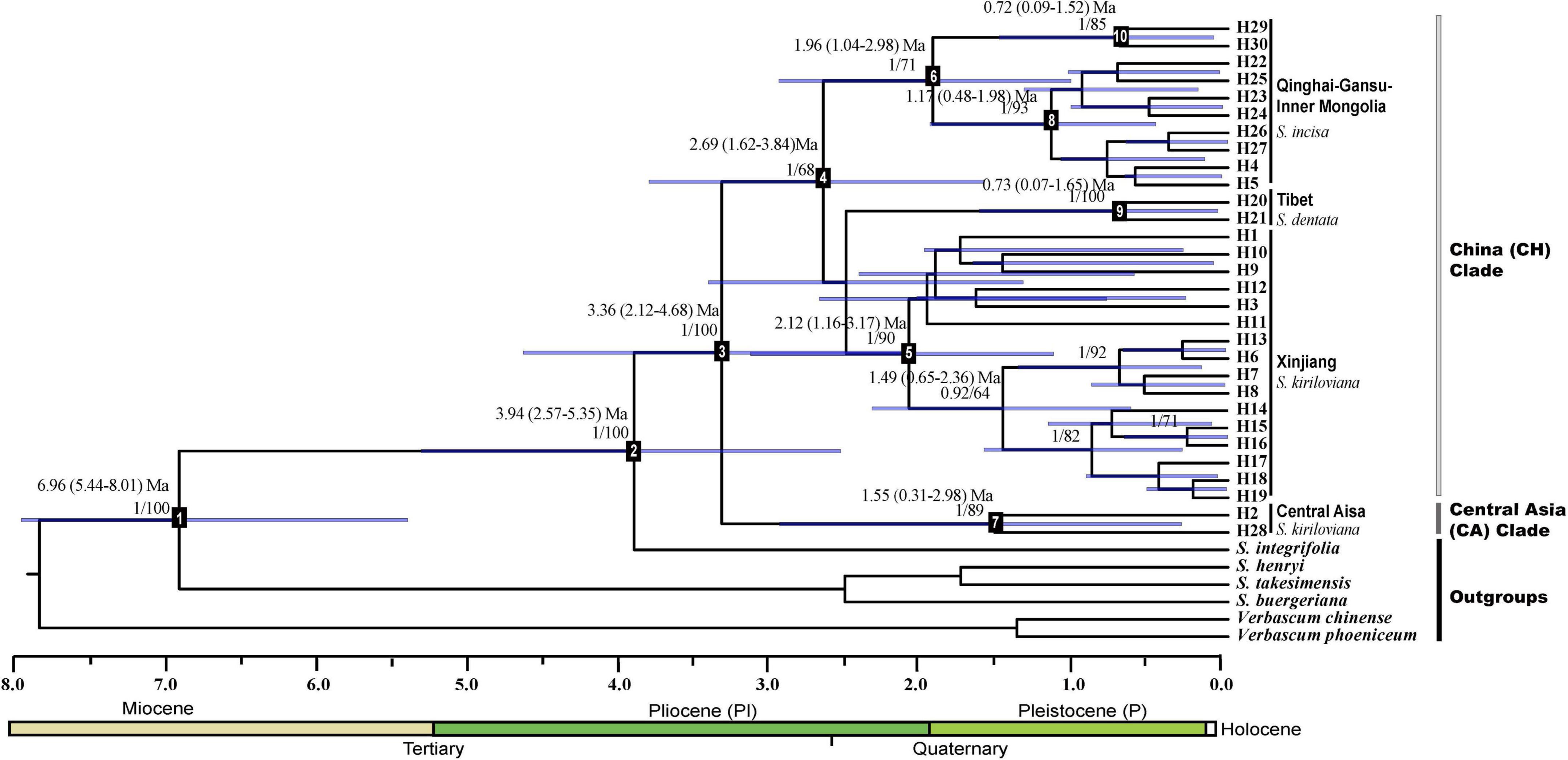
Figure 2. BEAST-derived chronograms of the Scrophularia incisa complex based on cpDNA (trnL–trnF, psbA–trnH, and trnQ–rps16). Blue bars indicate the 95% highest posterior density (HPD) credibility intervals for node ages (in Myr ago, Ma). Posterior probabilities and bootstrap values (>50%) based on maximum-likelihood (ML) analysis are sequentially labeled above nodes. Mean divergence dates and 95% HPDs for major nodes (1–10) are summarized in Table 2. Haplotypes are indicated by letter codes (H1–30).
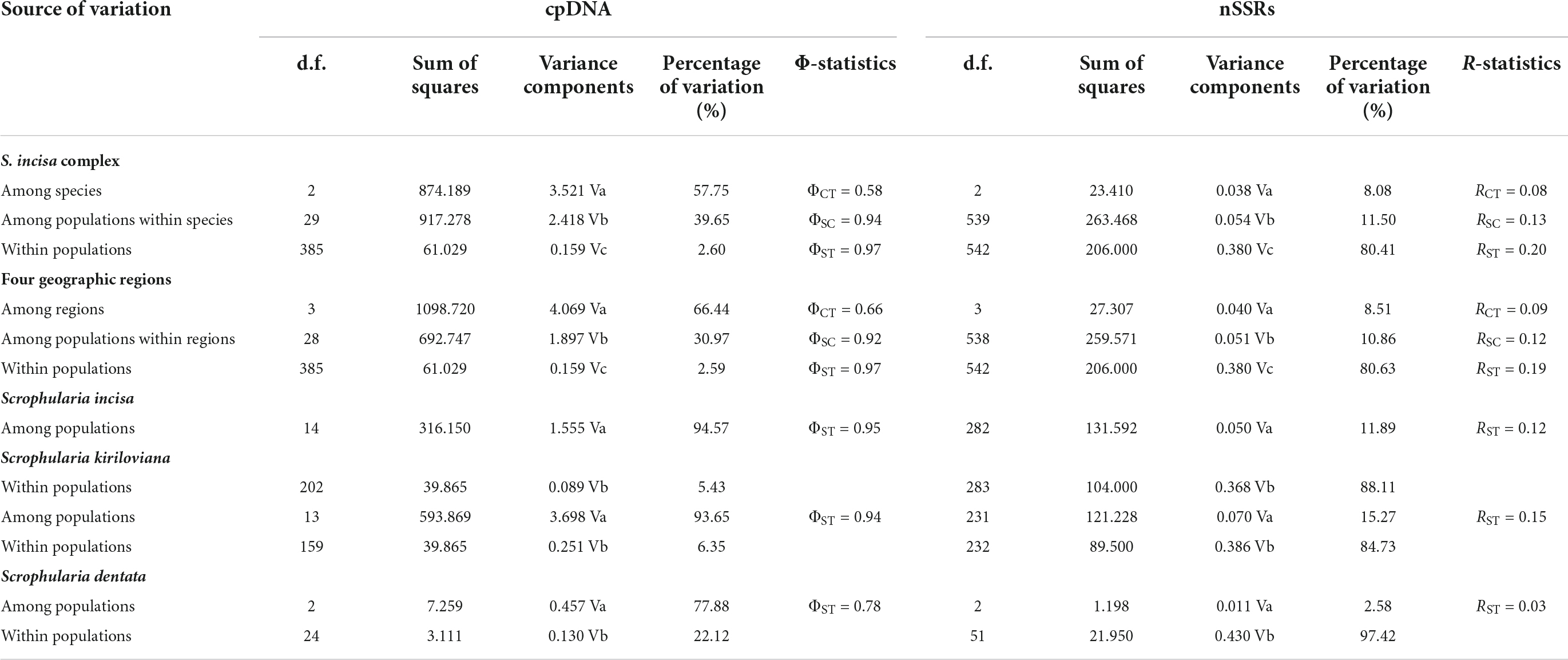
Table 1. The analysis of molecular variance (AMOVA) for cpDNA data and nSSR data among geographic regions and populations of Scrophularia incisa complex.
Ecological niche modelings and niche identity tests
Ecological niche models (ENMs) were developed for each species in MAXENT (Phillips et al., 2006) to predict suitable climate envelopes. A total of 78 collection records for S. incisa, 60 for S. kiriloviana, and 49 for S. dentata were obtained from the Chinese Virtual Herbarium1 and the National Specimen Information Infrastructure of China2 and Global Biodiversity Information Facility.3 Nineteen bioclimatic layers were downloaded from the WorldClim database4 (Hijmans et al., 2005) at 2.5 arc-min resolution, for current (the period 1960–1990), the LGM (c. 21,000 year before present; BP) predicted according to CCSM model and a future periods (2070) running the RCP8.5 experiment using the CCSM4 model, respectively. Highly correlated variables were identified using the “pairs” function of the R package RASTER v3.1-5 (Hijmans, 2020) and were removed to prevented potential overfitting, with five retained for analysis. These five variables with low correlation were BIO8 (mean temperature of wettest quarter), BIO10 (mean temperature of warmest quarter), BIO14 (precipitation of driest month), BIO17 (precipitation of driest quarter), BIO19 (precipitation of coldest quarter) for S. incisa, BIO3 (isothermality), BIO6 (min temperature of coldest month), BIO7 (temperature annual range), BIO8, BIO14 for S. dentata, and BIO3, BIO5 (max temperature of warmest month), BIO6, BIO11 (mean temperature of coldest quarter), BIO19 for S. kiriloviana. We performed 50 randomly subsampled replicate runs with 25% of observations retains for cross-validation. Models were further evaluated using Area Under the Curve (AUC) values of the Receiver Operating Characteristic (ROC) plot. AUC values greater than 0.9 indicates a very good prediction, while greater than 0.75 means the predicted model is better than a random model (Swets, 1988).
Niche identity tests were also performed to evaluate the niche similarity among the three species in ENMTools v1.4.3 (Warren et al., 2010) based on all the 19 BIOCLIM variables from the WorldClim dataset for testing the null hypothesis that S. incisa, S. kiriloviana, and S. dentata are occupying identical climatic environments (niches). Niche overlap was quantified using the standardized Hellinger dist enlargement in Northwesterance (I) and Schoener’s D (Schoener, 1968).
Ancestral area reconstructions
In order to reconstruct the geographical diversification of S. incisa complex, the Bayesian binary MCMC (BBM) analysis implemented in RASP v3.0 (Yu et al., 2020)5 was performed using trees retained from the interspecific BEAST analysis (see above). To prevent biased inferences toward wide or unlikely distributions for the crown node of the ingroup, caused by uncertainties regarding the root area of the outgroup (Migliore et al., 2012; Harris et al., 2013), we pruned one outgroup for our ancestral state reconstructions. Four geographic regions representing the current distribution were defined according to floristic divisions: A, Central Asia; B, Xinjiang; C, Qinghai-Gansu and Inner Mongolia; D, Tibet (Good, 1974; Meng et al., 2015). These floristic divisions are generally based on the floral composition and vegetation types (Meng et al., 2015). The number of maximum areas at each node was set to four. To account for phylogenetic uncertainty, 5,000 out of 20,000 post-burn-in trees from the BEAST analysis were randomly chosen for BBM analysis (see also Chen et al., 2014b). We set the root distribution to null, applied 10 MCMC chains with the JC + G model running for 106 generations, and sampled the posterior distribution every 100 generations.
Results
Chloroplast DNA diversity and population structure
The two cpDNA-IGS regions surveyed across the 413 individuals (33 populations) of the Scrophularia incisa complex were aligned, with a total length of 1,310 bp and 32 substitutions and 15 indels (Supplementary Table 4). In combination, these polymorphisms identified a total of 30 haplotypes (H1–30). Of those, 1 (H28) was specific to S. kiriloviana in Central Asia, 16 were specific to Xinjiang (H1, H3, H6–19) and H2 was shared between Central Asia and Xinjiang. Whereas eight were specific to S. incisa in Qinghai and Gansu (H4, H5, H22–27), two were specific to S. incisa in Inner Mongolia (H29–30) and two (H20, H21) were specific to S. dentata in Tibet. For the species complex as a whole, the cpDNA data revealed high levels of haplotype diversity (hT = 0.102) and nucleotide diversity (πT = 0.314 × 10–3). On average, S. kiriloviana had much higher levels of within-population diversity (hS = 0.132; πS = 0.302 × 10–3) than S. incisa (hS = 0.060; πS = 0.047 × 10–3), whereas the three S. dentata populations were fixed for H20 and H21 (Supplementary Table 1 and Figures 3A,B).
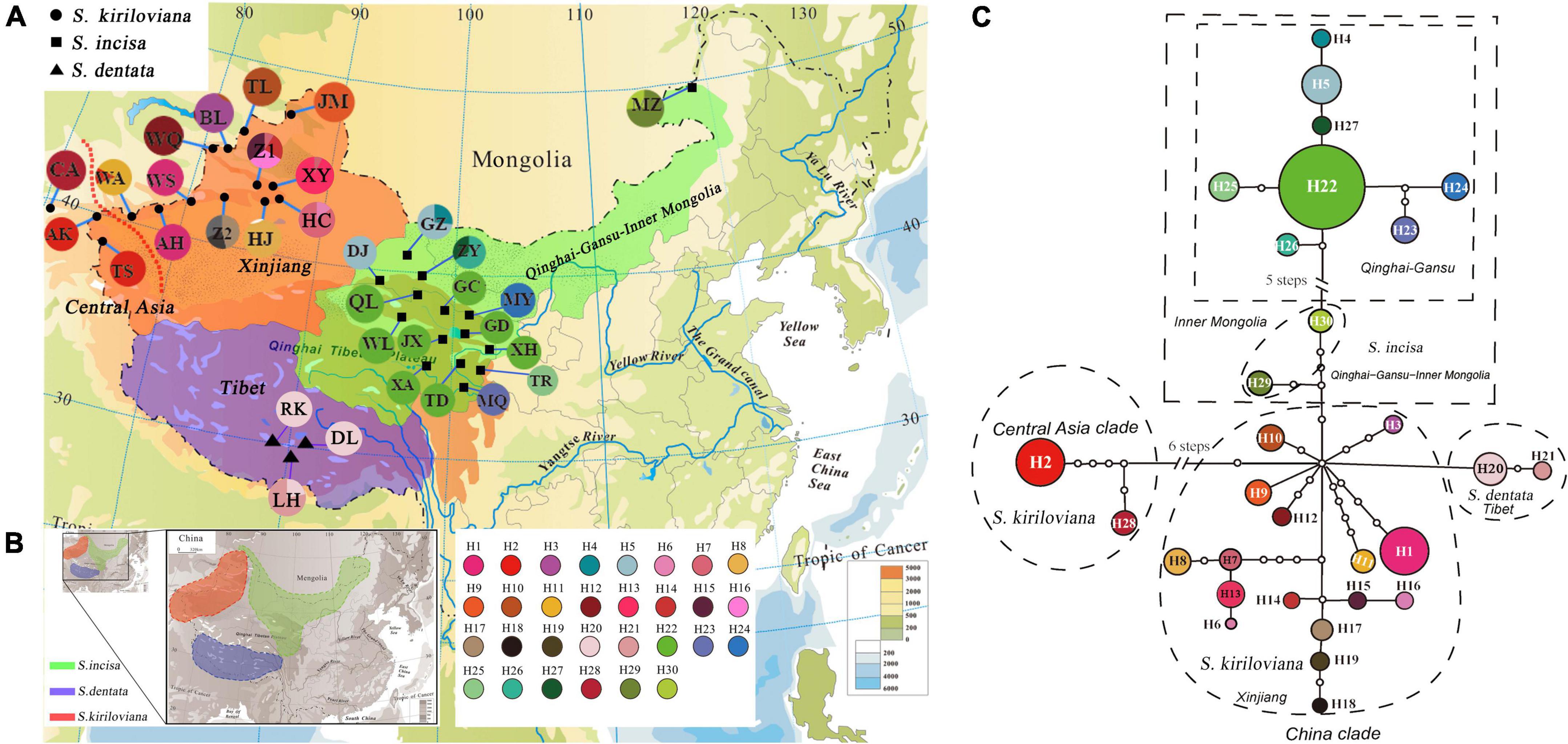
Figure 3. (A) Geographic distribution of the 30 chloroplast (cp) DNA (trnL-trnF and psbA-trnH) haplotypes (H1–H30) detected in the Scrophularia incisa complex (see Supplementary Table 1 for population codes). Red dashed lines denote divergences identified by TCS and phylogenetic analyses. (B) Geographic ranges of S. incisa, S. dentata and S. kiriloviana. (C) TCS-derived network of genealogical relationships of the 30 cpDNA haplotypes. The small open circles represent missing haplotypes. The size of circles corresponds to the haplotype frequency.
The parsimony network (Figure 3B) grouped the 30 cpDNA haplotypes into two major clades (Central Asia “CA” and China) separated by seven mutational steps. Thus, each region mostly harbored a genealogically distinct set of haplotypes except Central Asia and Xinjiang sharing H2. Within the China clade, haplotypes from Qinghai-Gansu (H4, H5, H22–27), Inner Mongolia (H29–30) and Tibet (H20–21) formed interior subclades relative to those from Xinjiang (H1, H3, H6–19) (Figures 3A,B). Hierarchical AMOVAs (Table 1) apportioned 57.75% of the total cpDNA variance among the three species, with 39.65% explained by variation among populations within species; in addition, 66.44% of this total variance distributed among four geographic regions (Central Asia, Xinjiang, Qinghai-Gansu-Inner Mongolia, Tibet) with 30.97% among populations within regions. Non-hierarchical analyses revealed stronger population genetic structure in both S. incisa (ΦST = 0.95) and S. kiriloviana (ΦST = 0.94) compared to S. dentata (ΦST = 0.78) (Table 1). A significant cpDNA phylogeographic structure was indicated for the whole species complex (NST = 0.966 > GST = 0.870), S. kiriloviana (NST = 0.915 > GST = 0.843) and S. incisa (NST = 0.982 > GST = 0.877) (all P < 0.05), but not for S. dentata (NST and GST = 0.75).
Phylogenetic haplotype relationships and molecular dating
The cpDNA tree topologies obtained from Bayesian inference (BI) (Figure 2) and maximum likelihood (ML, not shown) supported the monophyly of both the S. incisa complex (posterior probability, PP = 1, ML bootstrap support = 100%) with S. integrifolia as the sister group (PP = 1, ML = 100%) and its divisions into two well-supported main lineages: Central Asia clade (“CA”; PP = 1, ML = 89%) and China clade (PP = 1, ML = 68%). The CA clade contained haplotypes of S. kiriloviana from the eastern foot of the Pamirs Plateau (pops. AK, TS, WA: H2) in Southwest of Xinjiang and West Tien-Shan (pop. CA: H28; Uzbekistan) in Central Asia. Within the China clade, three lineages were resolved: (i) a strongly supported “Xinjiang” clade (PP = 1, ML = 90%) comprising all the remaining haplotypes of S. kiriloviana; (ii) a strongly supported “Tibet” clade (PP = 1, ML = 100%), consisting of all S. dentata haplotypes; (iii) a well-supported “Qinghai-Gansu–Inner Mongolia” clade (“QGI”), containing all haplotypes of S. incisa (PP = 1, ML = 71%), which could be further divided into Qinghai-Gansu (PP = 1, ML = 93%) and Inner Mongolia (PP = 1, ML = 85%) subclades.
Based on our BEAST-derived chronogram (Figure 2), the S. incisa complex likely originated in the Middle Pliocene at c. 3.94 Ma (node 2) and started to diversify at c. 3.36 Ma (node 3), whereas the crown ages of the main lineages fell into the Late Pliocene to Middle Pleistocene (China: c. 2.69 Ma, node 4; Xinjiang: c. 2.12 Ma, node 5; QGI: c. 1.96 Ma, node 6; CA: c. 1.55 Ma, node 7; Tibet: c. 0.73 Ma, node 9) (Table 2). For this chronogram, BEAST provided an average substitution rate of 1.07 × 10–9 s/s/y, which is little slower than the mean values usually reported for non-coding cpDNA regions (e.g., 1.2–1.7 × 10–9 s/s/y; Graur and Li, 2000).
Demographic analyses based on chloroplast DNA sequence variation
Estimates of Tajima’s D and Fu’s Fs were generally non-significant for all cpDNA clades of the S. incisa complex (Table 3). By contrast, the observed mismatch distribution of haplotypes for each clade failed to reject the demographic expansion model in most cases (SSD, HRag values P > 0.01; Table 3). Nevertheless, only the mismatch distributions of the “Xinjiang” and “Qinghai-Gansu–Inner Mongolia” clades, were unimodal and general fit to the distributions expected under a demographic expansion model (Table 3 and Supplementary Figure 1). Based on the corresponding τ values, and assuming a substitution rate of 1.07 × 10–9 s/s/y (see above), we dated the two demographic expansions to the Early Pleistocene (Xinjiang: c. 0.832 Ma, 95% CI: 0.485–1.103 Ma) or Middle Pleistocene (Qinghai-Gansu-Inner Mongolia: c. 0.370 Ma, 95% CI: 0.172–0.683 Ma) (Table 3).

Table 3. Results of mismatch distribution analysis (MDA) and neutrality tests for pooled populations of clades of Scrophularia incisa complex.
Nuclear microsatellite genotyping, population structure, and migration/gene flow
Using FREENA, the frequency of null alleles at each of the 12 nSSR loci was lower than the threshold (ν = 0.15) across the 32 populations of the S. incisa complex. There was no evidence for LD and significant deviation from Hardy-Weinberg equilibrium. All populations revealed a high genetic diversity across the 12 loci surveyed, with mean per-locus estimates of allele and gene diversity of NA = 16.563 (range: 2–24) and total HS = 3.033, along with private gene diversity (PAR = 0.150), observed heterozygosity (HO = 0.782) and expected heterozygosity (HE = 0.774) (Supplementary Table 1). At the species level, measures of HE derived from all 12 loci were highest in S. incisa (0.782), followed by S. kiriloviana (0.771) and S. dentata (0.685). In the hierarchical AMOVA, 8.08% of the total nSSR variation was distributed among the three species (RCT = 0.08), 11.50% was explained by variation among populations within species (RSC = 0.13), and 80.41% was apportioned within populations (RST = 0.20; Supplementary Table 1). Non-hierarchical analyses revealed less strong population genetic structure in S. incisa (RST = 0.12), S. kiriloviana (RST = 0.15), and S. dentata (RST = 0.03) (Supplementary Table 1).
For the entire nSSR dataset (32 populations, n = 522), STRUCTURE yielded the highest likelihood when samples were clustered into three groups (K = 3). All individuals of S. kiriloviana from Xinjiang and S. incisa from Inner Mongolia formed a separate cluster (“red” in Figure 4), whereas those of S. incisa from Qinghai and Gansu were assigned to the “green” gene pool; by contrast, all individuals of S. dentata from Tibet formed the third (“blue”) cluster. The nSSR dataset thus showed a geographic distribution pattern that was largely congruent with that of cpDNA (Figure 3), apart from a population of S. incisa from Inner Mongolia (population MZ), which form a monophyly cpDNA lineage with S. incisa from Qinghai-Gansu, but fall into S. kiriloviana “red” gene pool according to nSSR result.
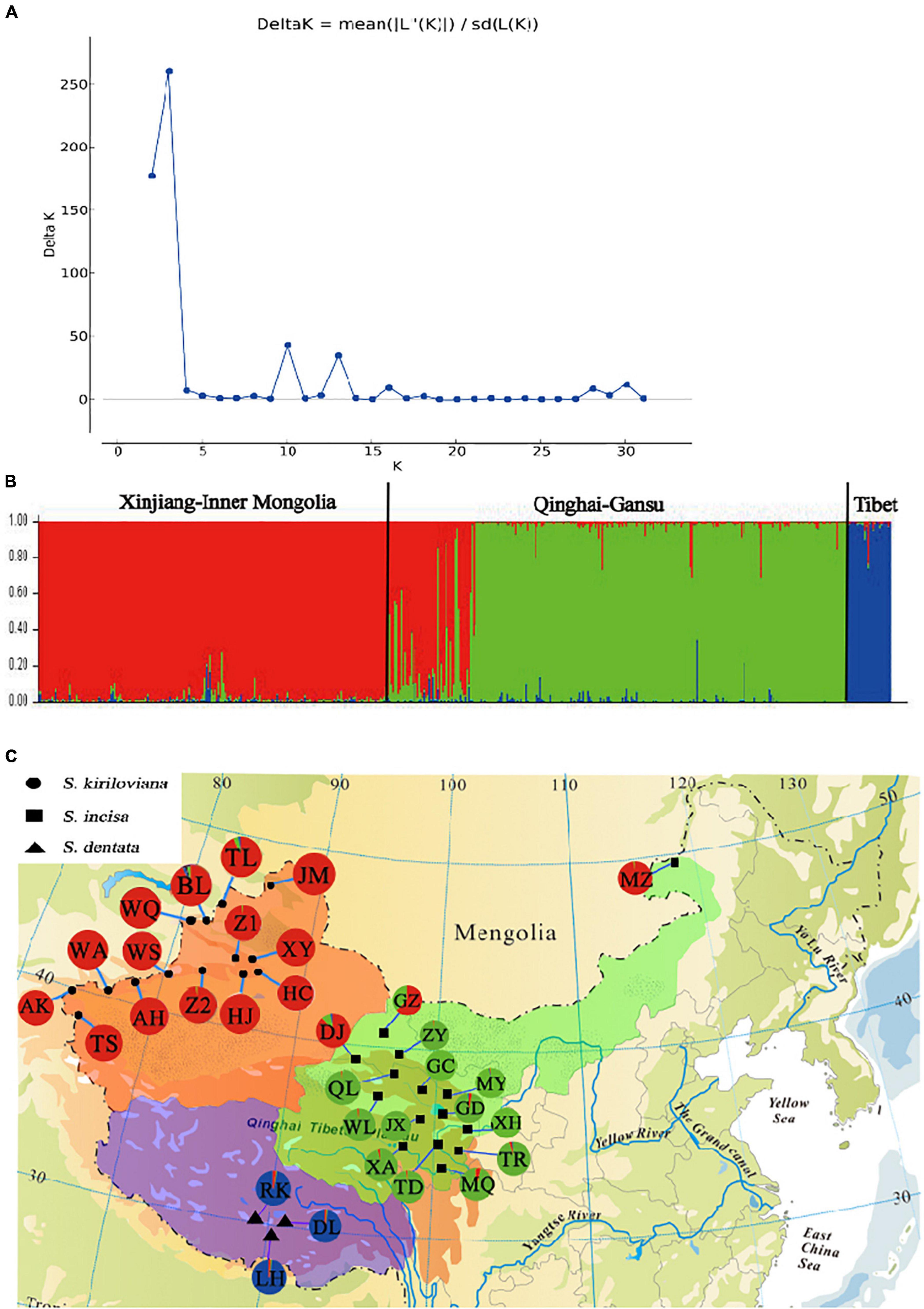
Figure 4. Bayesian clustering results of the STRUCTURE analysis for nSSR data of 530 individuals (32 populations) of Scrophularia incisa complex from Northwest China. Number of clusters (K) was varied from 1 to 32 in 10 independent runs. (A) The dot plot represents changes of the mean posterior probability [loge P(D)] (±SD) values of each K calculated according to Pritchard et al. (2000), whereas the superimposed line diagram indicates the corresponding ΔK statistics calculated according to Evanno et al. (2005). (B) Histogram of the STRUCTURE analysis for the model with K = 3 (showing the highest ΔK). The smallest vertical bar represents one individual. The assignment proportion of each individual into one of three population clusters (or “gene pools”) is shown along the y-axis. (C) Geographic origin of the 32 S. incisa complex populations and their color-coded grouping according to the STRUCTURE analysis. Population codes are identified in Supplementary Table 1.
Based on the nSSR data, all 12 pairwise estimates of inter-region migration rates were significant (no 95% confidence intervals overlapping zero), ranging from 3.426 (Tibet to Xinjiang) to 10.920 (Xinjiang to Tibet). And it’s also, for instance, the most distinctly obvious asymmetrical pair of migration rates for S. incisa complex. There was also relatively high gene flow from Qinghai-Gansu-Inner Mongolia to Tibet (8.9710) and, to a lesser extent, in the reverse direction (4.4978). In general, estimates of inter-region gene flow were of low magnitude and mostly symmetrical (Table 4).

Table 4. Estimates of migration rate (M) and 95% confidence intervals (CI) (in parentheses) between four Scrophularia incisa complex geographic regions using MIGRATE.
Predicted distributions and niche identity tests
The constructed ecological niche models performed well for S. incisa complex with high AUC scoring above 0.96, indicating good predictive model performance. The most important environmental predictors were precipitation of driest month (BIO14) for S. dentata, and precipitation of coldest quarter (BIO19) for S. incisa and S. kiriloviana. The species’ predicted potential distribution of the species complex under current conditions (1950–2000) was generally similar to their actual distributions (Figure 5). Suitable habitat for S. incisa at the LGM contracted greatly in the northeastern part of QTP, whereas S. dentata retreated to southern and western parts of the Himalaya Mountains, and S. kiriloviana became mainly restricted to the Tianshan Mountains (Figure 5). Suitable habitat for S. dentata and S. kiriloviana is predicted to become much larger by 2070 with a shift eastwards and expansion northwards and southwards from the Tianshan Mountains, respectively; however, for this future scenario, suitable habitat for S. incisa is predicted to become fragmented and extinct in Ningxia and the Midwest of Inner Mongolia (Figure 5).
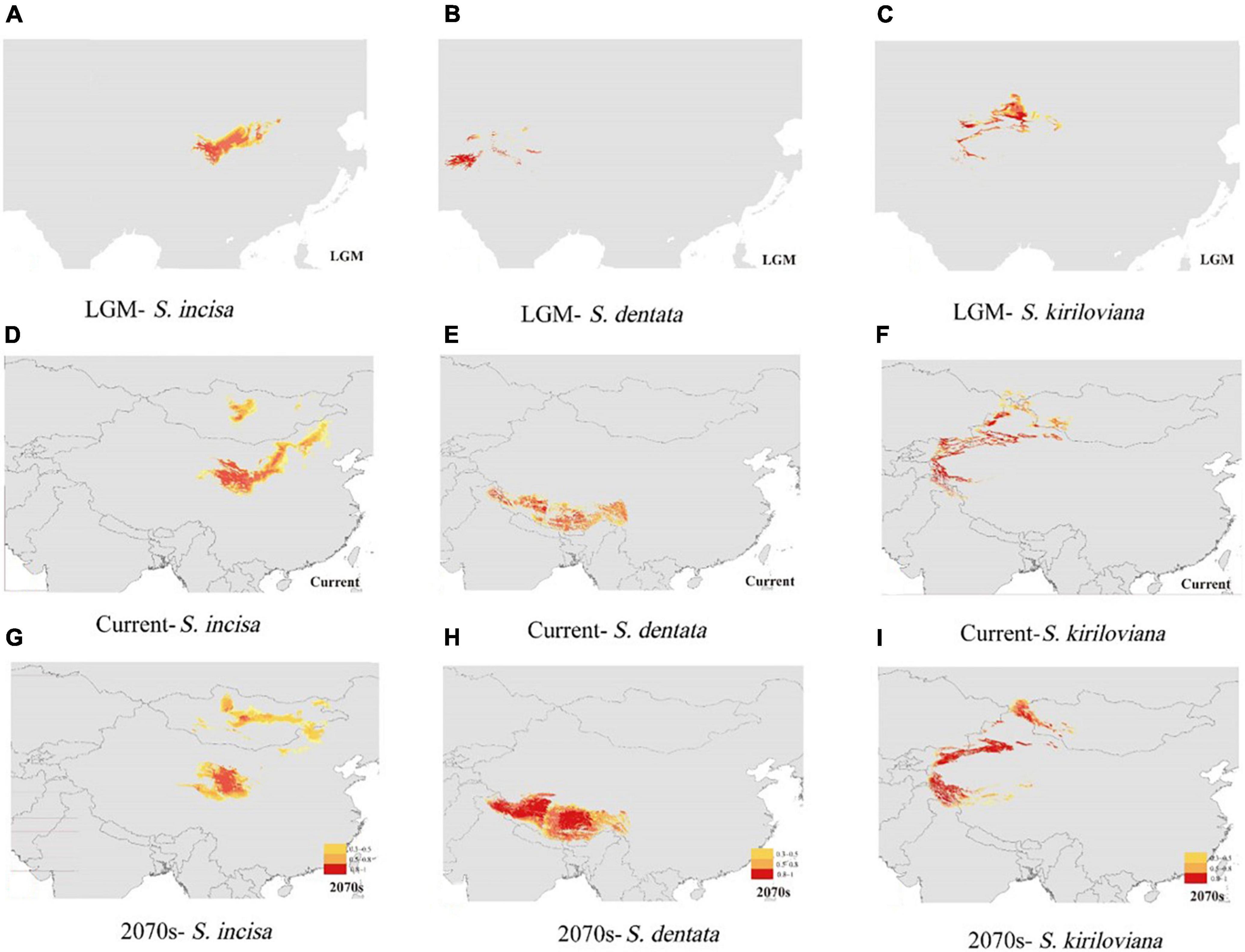
Figure 5. Predicted distributions of S. incisa, S. dentata, and S. kiriloviana at the (A–C) Last Glacial Maximum (LGM; 21 000 year before present; BP), under (D–F) current conditions (1950–2000), and (G–I) future (2070s) based on ecological niche modeling using MAXENT v3.3.1 (Phillips et al., 2006). Maps were generated using ARCGIS v9.3 (ESRI, Redlands, CA, USA).
The results of the niche identity tests undertaken using ENMTools supported the existence of niche differentiation among the three species, the observed values of Schoener’s D and Hellinger’s I (between S. incisa and S. kiriloviana: 0.296 and 0.535; between S. dentata and S. incisa: 0.264 and 0.476; between S. dentata and S. kiriloviana: 0.267 and 0.503, respectively) were significantly lower than the null distributions, indicating that S. incisa, S. kiriloviana and S. dentata were ecologically distinct species (Supplementary Table 5).
Ancestral area reconstructions
Based on the topology of the BEAST chronogram (Figure 2), the BBM analysis of ancestral distribution areas (Figure 6) supported a probably ancient (pre-Quaternary) distribution of the S. incisa complex in Central Asia (node I). A vicariant event, evident at this node, was likely followed by two independent colonization events from Central Asia (node II) to Xinjiang (B) and Qinghai-Gansu–Inner Mongolia (C), respectively, possibly during the Late Pliocene to Early Pleistocene (see nodes 4, 5, 6 in Figure 2), then followed by a more recent (Pleistocene) dispersal event from Xinjiang (B) to Tibet (D: H20, H21) (see Figure 6); geographical vicariance between these regions was established.
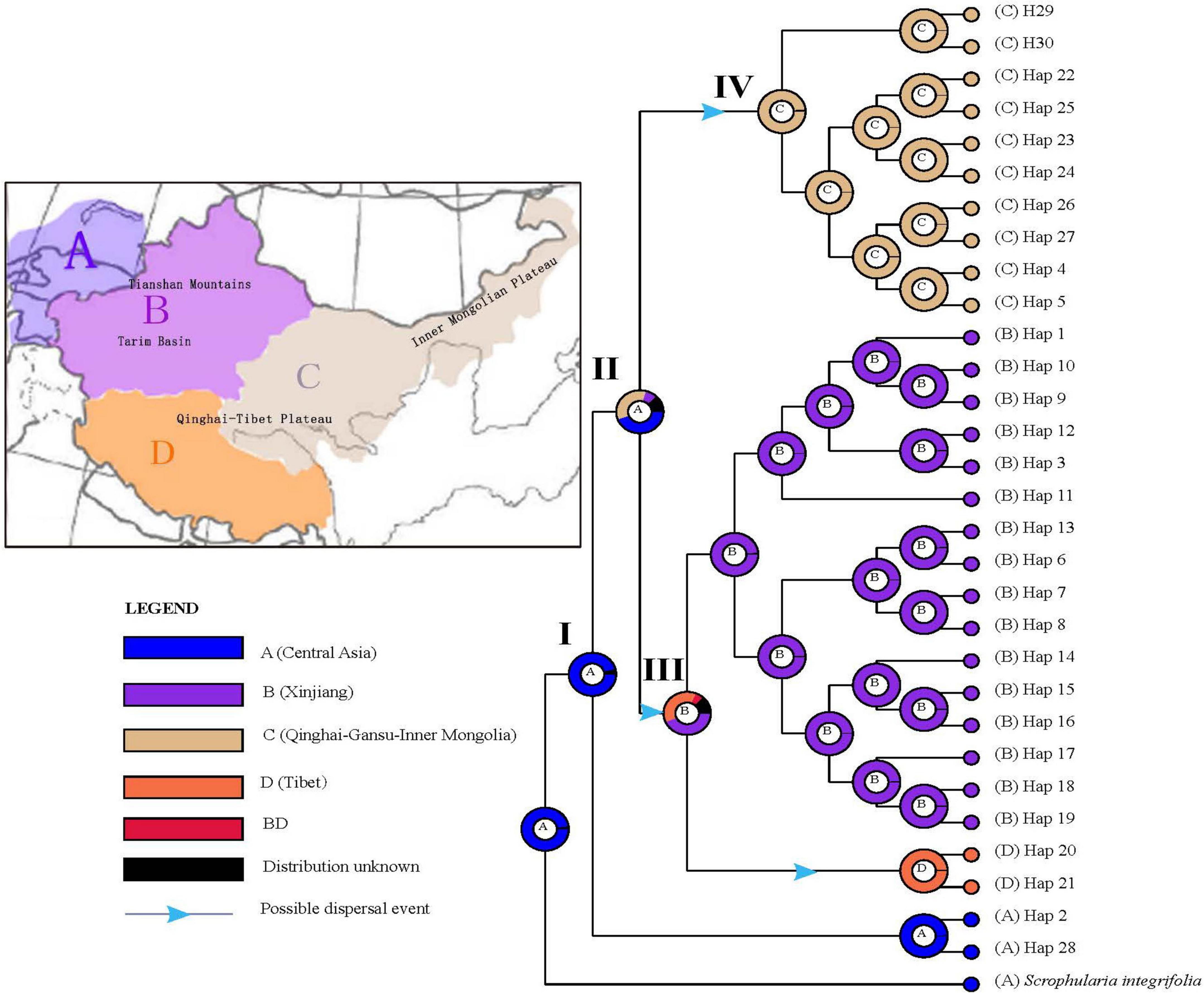
Figure 6. Ancestral area reconstructions based on the Bayesian binary Markov chain Monte Carlo (BBM) method implemented in RASP using the BEAST-derived chronogram of Scrophularia incisa complex (see Figure 2). The insert map shows major floristic divisions (A–D). Pie charts of each node illustrate the marginal probabilities for each alternative ancestral area derived from BBM with the maximum area number set to four. The color key identifies possible ancestral ranges at different nodes. Possible dispersal events are indicated by blue arrows.
Discussion
Species boundaries and cytoplasmic-nuclear discordance in Scrophularia incisa complex
Our cpDNA haplotype network (Figure 3) and phylogeny (Figure 2) indicate that the “Central Asia” (CA) clade of S. kiriloviana mainly occurs along the eastern foothills of the Pamirs Plateau in Southwest Xinjiang and west of the Tianshan Mountains in Uzbekistan; by contrast, the “China” (CH) clade consists of three subclades in Xinjiang (S. kiriloviana), Tibet (S. dentata) and Qinghai-Gansu–Inner Mongolia (S. incisa), respectively. Based on the nSSR data (Figure 4), the S. incisa complex is divided into three gene pools, Xinjiang–Inner Mongolia (S. kiriloviana and S. incisa), Qinghai-Gansu (S. incisa), and Tibet (S. dentata), respectively. Hence, this nuclear dataset identifies a similar broad-scale phylogeographic pattern across the complex, as registered by cpDNA, except for a population (MZ) of S. incisa from Inner Mongolia fell into the Xinjiang gene pool (Figure 4). Similarly, shared genotypes between the clades were not found in their cpDNA sequence, but appeared in the nSSR datasets. Some individuals in the two populations (GZ and DJ) in northwestern Gansu show dominant red genetic clusters from Xinjiang gene pool and the rest populations from Qinghai-Gansu show little red genetic cluster from Xinjiang gene pool and blue genetic cluster from Tibet (Figure 4). The same situation to populations form Xinjiang and Tibet clade that have more or less genetic clusters from other gene pools, which maybe due to the mostly low magnitude of symmetrical inter-region gene flow (Table 4). We suggest that there are three recognized species, and several examples of incongruence between the cpDNA and nSSR data sets indicate that introgression had occurred among the three species, and/or lineage sorting between different groups was incomplete (Wang et al., 2008; Lin et al., 2019).
Allopatric divergence and evolution history
Our cpDNA results show that the S. incisa complex is comprised of two major clades [Central Asia (CA) vs. China], with unique sets of haplotypes and distinct geographic distributions (Figure 3). In conjunction with BEAST-derived our molecular dating (Figure 2), the ancestral range reconstructions (Figure 6) indicate that the split of CA and China clades during the Middle Pliocene at c. 3.36 (2.12–4.68) Ma. Subsequently, the China clade is further divided into four subclades, namely, “Xinjiang” (c. 2.12), “Tibet” (c. 0.73), “Qinghai-Gansu” (c. 1.17), and “Inner Mongolia” (c. 0.72), respectively (Figure 2). Most studies support species divergences in the last 5 Ma, such as in the genera of Rheum L., Sinacalia H. Rob. and Brettell and Solms-laubachia (Maxim.) Botsch (Wang et al., 2005; Liu et al., 2006; Yue et al., 2009), except some plant lineages diverged following the early uplifts of the QTP, or even prior to the formation of the plateau (Liu et al., 2002; Yang et al., 2003; Zhang and Fritsch, 2010; Sun et al., 2012). However, extensive heterogeneous uplift events across the QTP sensu lato (sl) which have occurred from the Miocene to Pliocene according to available evidence (Manish and Pandit, 2018; Fang et al., 2020; Mao et al., 2021), in association with a monsoon-dominated climate pattern (An et al., 2001; Guo et al., 2008; Spicer, 2017) and global climate cooling (Shackleton et al., 1995; Zachos et al., 2001) triggered aridification increasement and sand deserts enlargement in Northwestern China (Sun et al., 2008; Miao et al., 2012). These climatic and geological changes may have intensified the vicariance and fragmentation, which played a key role in plant morphogenesis and adaptive evolution (Xu et al., 2022), further facilitated the plant allopatric speciation and divergence into different lineages and species (Qiu et al., 2011; Li et al., 2012; Wen et al., 2016). This inference of eco-geographically driven, and possible adaptive divergence is further illustrated by our niche modeling, which indicates that the three species occupy significantly different climatic environments (Supplementary Table 5).
Underlying reasons of how organisms are spread geographically is a core objective of biogeography (Cox and Moore, 2000). Reviewing recent evidences from phylogenetic and biogeographic studies in plants, the Tethyan Tertiary flora and the Arcto-Tertiary flora have been indicated as important sources of the QTP alpine and forest floras (Sun, 2002; Liao et al., 2012; Zhou et al., 2013; Wen et al., 2014). Some others suggested that majority of these temperate groups originated on the QTP and subsequently migrated into the other regions of Eurasia, during global temperature decreased and the QTP uplifted extensively, i.e., the out-of-QTP hypothesis (Ozenda, 1988; Jia et al., 2012; Fan et al., 2013; Zhang J. Q. et al., 2014). CpDNA haplotype variation phylogenetic tree demonstrated that the basal phylogenetically clades of the S. incisa complex were exclusively distributed in the Central Asia (Figure 2), supporting the hypothesis of “migrations/dispersals from Central Asia into the QTP,” as inferred from various genera, such as Solms-laubachia, Incarvillea, and Myricaria (Grierson, 1961; Chen et al., 2005; Wang et al., 2009; Yue et al., 2009). Further evidence of this hypothesis came from RASP-BBM analysis of the cpDNA tree (Figure 6), which suggested that Central Asia, cradle of the S. incisa complex and multiple dispersals from Central Asia accounted for most of the species’ range expansion in Asia. The inferred migration route of S. incisa complex to China through intervening mountain ranges in northwestern Himalaya is one suggested previously for many plants of the QTP and adjacent regions that originated in the Central Asia region (Yue et al., 2009). Another corridor from Central Asia via the north of Xinjiang to Mongolia Plateau, Qinghai and Gansu is also reported before (Chen et al., 2005; Jia et al., 2012). It was estimated that two dispersal routes to QTP via northwestern Himalaya to Tibet and via northern Xinjiang to Inner Mongolia, Qinghai and Gansu with both dispersal events having their source in Central Asia. The phylogeographic patterns based on the maternally inherited cpDNA are likely to reflect past migration of the species through long-distance seed dispersal mediated by river. According to our field investigation, the S. incisa complex is usually distributed along the alpine water of the melt snow in the valley, floodplains, and streams, we speculate the seeds in capsule propagate by river.
Glacial refugia, habitat fragmentation, and conservation implications
Among the remarkable climatic changes of the Cenozoic, the climatic oscillations during the Quaternary had great influence on the genetic structure and distribution of extant plants in China (Comes and Kadereit, 1998; Hewitt, 2000; Wen et al., 2016). Species experienced glacial-time retreat to separate refugia and interglacial recolonization in response to cold-warm climatic cycles (Hewitt, 2000), separate refugia are hypothesized to result in fragmentation of the geographical distributions, deep allopatric divergence and intraspecific differentiation, especially the plants in the Altay-Tianshan Mountains (Tang et al., 2010; Wu et al., 2010; Meng et al., 2015). Our genetic and ENM evidence for S. kiriloviana in Xinjiang and S. incisa in Qinghai-Gansu have shown significant phylogeographical structure with the location of refugia in the Altay-Tianshan Mountains and southeast of Qinghai Plateau, as considered the biotic glacial refugium where plants persisted during glacial periods (Meng et al., 2015). The humid valleys from Altay-Tianshan Mountains may provide refugial habitats for S. kiriloviana, while the distribution of most our sampled populations of S. kiriloviana from Xinjiang were fragmented in different valleys. S. incisa in Qinghai-Gansu occurring on the plateau platform was speculated to experience postglacial expansion and recolonization from the refugia located at lower elevations at the southeast plateau edge (Li et al., 2011; Qiu et al., 2011), although the expansion signal pertaining to the Qinghai-Gansu-Inner Mongolia clade most likely occurred before the LGM and cannot be linked to a major postglacial demographic event, which maybe due to the influence of population from Inner Mongolia on the demographic analysis and expansion time estimation of Qinghai-Gansu populations. Moreover, as our ENM results predict fragmented distribution for S. incisa and extinction in Ningxia and midwestern Inner Mongolia by 2070 (Figure 5), which is consistent with our field investigations that its current population number and size appears limited in midwestern Inner Mongolia, possibly as a consequence of over-exploitation and habitat destruction by human activity for its medicinal value (Guo et al., 2019; Han et al., 2022), and none individuals were collected during our fieldwork. So we suggest that in situ conservation efforts should be made such as establishing protected areas and population recovering in natural habitat for S. incisa (Zhou et al., 2021).
Conclusion
Our phylogeographic and niche modeling evidences suggest that there are three genetically and ecological recognized species, comparison of the topologies of cpDNA network/tree and nSSR structure revealed several examples of incongruence indication that historical hybridization or incomplete lineage sorting had occurred among the three species and between different clades. The S. incisa complex diverged at the molecular level into several distinct clades, as resolved in the cpDNA phylogenetic tree and network. Our molecular dating revealed that the origin of these clades dates back to the Middle Pliocene, c. 3.94 Ma, when the QTP and other parts of Eurasia underwent considerable geological change and/or climatic oscillations. Such paleo-events are likely to have fragmented the distribution of the complex and triggered allopatric divergence and the formation of deep clades. Our phylogenetic based AAR evidences support that S. incisa complex originated in the Central Asia with subsequent dispersals from the Central Asia into Northwestern China and diversification of several groups in the Tianshan Mountains, the QTP and the Inner Mongolian Plateau. This work therefore provides a further example of the overriding role of the Plio-Pleistocene climate change, in this case aridification triggering allopatric speciation and diversification in this group of steppe and desert plants of Northwestern China.
Data availability statement
The cpDNA sequences data obtained in this study are deposited in GenBank (see Supplementary Table 2 for accession number). Microsatellite genotypes and maxent input file are openly available in Github at https://github.com/ruihongwang77/Microsatellite-genotypes-and-maxent-data-of-Scrophularia-incisa-complex.
Author contributions
C-XF conceived and designed the study. PL, C-XF, R-HW, and Z-PY collected the samples. R-HW performed the experiments, analyzed the data, and wrote drafts of the manuscript. Z-CZ participated in the data analysis. HC and Z-CQ helped to improve the final manuscript. All authors contributed to the article and approved the submitted version.
Funding
This research was supported by the National Nature Science Foundation of China (31600183, 31871694, and 31070205), the Opening Project of Xinjiang Production & Construction Corps Key Laboratory of Protection and Utilization of Biological Resources in Tarim Basin (BRZD1303), the Natural Science Foundation of Zhejiang Province (LGH22H280005 and LY21C030008), the Science Foundation of Zhejiang Sci-Tech University (15042170-Y), and the Fundamental Research Funds of Zhejiang Sci-Tech University (2020Q031).
Acknowledgments
We thank N. Chen and S. D. Shi for help in the field plant materials collecting, J. J. Wu and L. L. Ding for help with the ENM analysis and geographic distribution map drawing, respectively.
Conflict of interest
The authors declare that the research was conducted in the absence of any commercial or financial relationships that could be construed as a potential conflict of interest.
Publisher’s note
All claims expressed in this article are solely those of the authors and do not necessarily represent those of their affiliated organizations, or those of the publisher, the editors and the reviewers. Any product that may be evaluated in this article, or claim that may be made by its manufacturer, is not guaranteed or endorsed by the publisher.
Supplementary material
The Supplementary Material for this article can be found online at: https://www.frontiersin.org/articles/10.3389/fpls.2022.985372/full#supplementary-material
Footnotes
- ^ www.cvh.ac.cn
- ^ www.nsii.org.cn
- ^ www.gbif.org
- ^ http://www.worldclim.org
- ^ http://mnh.scu.edu.cn/soft/blog/RASP
References
Abbott, R. J., Smith, L. C., Milne, R. I., Crawford, R. M. M., Wolff, K., and Balfour, J. (2000). Molecular analysis of plant migration and refugia in the Arctic. Science 289, 1343–1346. doi: 10.1126/science.289.5483.1343
An, Z. S., John, E. K., Warren, L. P., and Stephen, C. P. (2001). Evolution of Asian monsoons and phased uplift of the Himalaya-Tibetan Plateau since late Miocene times. Nature 411, 62–66. doi: 10.1038/35075035
Avise, J. C. (2000). Phylogeography: The history and formation of species. London: Harvard University Press.
Avise, J. C. (2009). Phylogeography: Retrospect and prospect. J. Biogeogr. 36, 3–15. doi: 10.1111/J.1365-2699.2008.02032.X
Beerli, P. (2006). Comparison of Bayesian and maximum-likelihood inference of population genetic parameters. Bioinformatics 22, 341–345. doi: 10.1093/bioinformatics/bti803
Beerli, P., and Felsenstein, J. (2001). Maximum likelihood estimation of a migration matrix and effective population sizes in n subpopulations by using a coalescent approach. Proc. Natl. Acad. Sci. U.S.A. 98, 4563–4568. doi: 10.1073/pnas.081068098
Bernatchez, L. (2001). The evolutionary history of brown trout (Salmo trutta L.) inferred from phylogeographic, nested clade, and mismatch analyses of mitochondrial DNA variation. Evolution 55, 351–379. doi: 10.1554/0014-38202001055[0351:TEHOBT]2.0.CO;2
Chen, C., Li, P., Wang, R. H., Schaal, B. A., and Fu, C. X. (2014a). The Population genetics of cultivation: Domestication of a traditional Chinese medicine, Scrophularia ningpoensis Hemsl. (Scrophulariaceae). PLoS One 9:e105064. doi: 10.1371/journal.pone.0105064
Chen, C., Qi, Z. C., Xu, X. H., Comes, H. P., Koch, M. A., Jin, X. J., et al. (2014b). Understanding the formation of Mediterranean–African–Asian disjunctions: Evidence for Miocene climate-driven vicariance and recent long-distance dispersal in the Tertiary relict Smilax aspera (Smilacaceae). New Phytol. 204, 243–255. doi: 10.1111/nph.12910
Chen, S., Guan, K., Zhou, Z., Olmstead, R., and Cronk, Q. (2005). Molecular phylogeny of Incarvillea (Bignoniaceae) based on ITS and trnL–F sequences. Am. J. Bot. 92, 625–633. doi: 10.3732/ajb.92.4.625
Clement, M., Posada, D., and Crandall, K. A. (2000). TCS: A computer program to estimate gene genealogies. Mol. Ecol. 9, 1657–1659. doi: 10.1046/j.1365-294x.2000.01020.x
Comes, H. P., and Kadereit, J. W. (1998). The effect of quaternary climatic changes on plant distribution and evolution. Trends Plant Sci. 3, 432–438. doi: 10.1016/S1360-1385(98)01327-2
Cox, C. B., and Moore, P. D. (2000). Biogeography: An ecological and evolutionary approach. Oxford: Blackwell Science. doi: 10.1086/628353
Cun, Y. Z., and Wang, X. Q. (2010). Plant recolonization in the Himalaya from the southeastern Qinghai-Tibetan Plateau: Geographical isolation contributed to high population differentiation. Mol. Phylogenet. Evol. 56, 972–982. doi: 10.1016/j.mpev.2010.05.007
Ding, Z. L., Derbyshire, E., Yang, S. L., Sun, J. M., and Liu, T. S. (2005). Stepwise expansion of desert environment across northern China in the past 3.5 Ma and implications for monsoon evolution. Earth Planet. Sci. Lett. 237, 45–55. doi: 10.1016/j.epsl.2005.06.036
Drummond, A. J., and Rambaut, A. (2007). BEAST: Bayesian evolutionary analysis by sampling trees. BMC Evol. Biol. 7:214. doi: 10.1186/1471-2148-7-214
Drummond, A. J., Ashton, B., Cheung, M., Heled, J., Kearse, M., Moir, R., et al. (2009). Geneious Pro V4.8.5. Available online at: http://www.geneious.com (accessed February 27, 2012).
Drummond, A. J., Nicholls, G. K., Rodrigo, A. G., and Solomon, W. (2002). Estimating mutation parameters, population history and genealogy simultaneously from temporally spaced sequence data. Genetics 161, 1307–1320. doi: 10.1093/GENETICS/161.3.1307
Eaton, D. A. R., Fenster, C. B., Hereford, J., Huang, S. Q., and Ree, R. H. (2012). Floral diversity and community structure in Pedicularis (Orobanchaceae). Ecology 93, S182–S194. doi: 10.1890/11-0501.1
Evanno, G., Regnaut, S., and Goudet, J. (2005). Detecting the number of clusters of individuals using the software structure: A simulation study. Mol. Ecol. 14, 2611–2620. doi: 10.1007/s10592-012-0338-x
Excoffier, L., and Lischer, H. E. (2010). Arlequin suite ver 3.5: A new series of programs to perform population genetics analyses under Linux and Windows. Mol. Ecol. Resour. 10, 564–567. doi: 10.1111/j.1755-0998.2010.02847.x
Fan, D. M., Chen, J. H., Meng, Y., Wen, J., Huang, J. L., and Yang, Y. P. (2013). Molecular phylogeny of Koenigia L. (Polygonaceae: Persicarieae): Implications for classification, character evolution and biogeography. Mol. Phylo. Evol. 69, 1093–1100. doi: 10.1016/j.ympev.2013.08.018
Fang, X. M., Dupont-Nivet, G., Wang, C. S., Song, C. H., Meng, Q. Q., Zhang, W. L., et al. (2020). Revised chronology of central Tibet uplift (Lunpola Basin). Sci. Adv. 6:eaba7298. doi: 10.1126/sciadv.aba7298
Fang, X. M., Shi, Z. T., Yang, S. L., Li, J. J., and Jiang, P. A. (2002a). Development of Gurbantunggut Desert and aridification of north Xinjiang recorded by Tianshan loss. Chin. Sci. Bull. 47, 540–545. doi: 10.1360/02tb9305
Fang, X. M., Lv, L. Q., Yang, S. L., Li, J. J., An, Z. S., Jiang, P. A., et al. (2002b). Loess in Kunlun Mountains and its implications on desert development and Tibetan Plateau uplift in west China. Sci. China Ser. D Earth Sci. 45, 289–299. doi: 10.3969/j.issn.1674-7313.2002.04.001
Fu, Y. X. (1997). Statistical tests of neutrality of mutations against population growth, hitchhiking and background selection. Genetics 147, 915–925. doi: 10.1093/GENETICS/147.2.915
Good, R. (1974). The geography of the flowering plants, 4th Edn. London: Longman. doi: 10.2307/1796048
Graur, D., and Li, W. H. (2000). Fundamentals of molecular evolution, 2nd Edn. Sunderland, MA: Sinauer Associates Inc. doi: 10.1046/j.1365-2540.2000.0728d.x
Grierson, A. J. C. (1961). A revision of the genus Incarvillea. Notes R. Bot. Gard. Edinb. 23, 303–354.
Guo, W. L., Yang, Z. Q., Hou, Z. N., Zhou, Z. Q., Qi, Z. C., Sun, Y. Q., et al. (2019). A comprehensive review of a Chinese folk herbal species Tetrastigmae hemsleyanum with multiplicity of pharmacological effects. Chin. Tradit. Med. J. 1, 1–19.
Guo, Z. T., Ruddiman, W. F., Hao, Q. Z., Wu, H. B., Qiao, Y. S., Zhu, R. X., et al. (2002). Onset of Asian desertification by 22 Myr ago inferred from loess deposits in China. Nature 416, 159–163. doi: 10.1038/416159a
Guo, Z. T., Sun, B., Zhang, Z. S., Peng, S. Z., Xiao, G. Q., Ge, J. Y., et al. (2008). A major reorganization of Asian climate by the early Miocene. Clim. Past 4, 153–174. doi: 10.5194/cp-4-153-2008
Han, Y. X., Pang, X. R., Zhang, X. M., Han, R. L., and Liang, Z. S. (2022). Resource sustainability and challenges: Status and competitiveness of international trade in licorice extracts under the Belt and Road Initiative. Glob. Ecol. Conserv. 34:e02014. doi: 10.1016/J.GECCO.2022.E02014
Harpending, H. C. (1994). Signature of ancient population growth in a low-resolution mitochondrial DNA mismatch distribution. Hum. Biol. 66, 591–600.
Harris, A. J., Wen, J., and Xiang, Q. Y. (2013). Inferring the biogeographic origins of intercontinental disjunct endemics using a Bayes-DIVA approach. J. Syst. Evol. 51, 117–133. doi: 10.1111/jse.12007
Hewitt, G. (2000). The genetic legacy of the Quaternary ice ages. Nature 405, 907–913. doi: 10.1038/35016000
Hewitt, G. M. (2004). Genetic consequences of climatic oscillations in the Quaternary. Philos. Trans. R. Soc. B Biol. Sci. 359, 183–195. doi: 10.1098/rstb.2003.1388
Hijmans, R. J. (2020). Raster: Geographic data analysis and modeling. R package version 3.1-5. Available online at: https://CRAN.R-project.org/package=raster (accessed April 19, 2020).
Hijmans, R. J., Cameron, S. E., Parra, J. L., Jones, P. G., and Jarvis, A. (2005). Very high resolution interpolated climate surfaces for global land areas. Int. J. Climatol. 25, 1965–1978. doi: 10.1002/joc.1276
Hong, D. Y. (1983). The distribution of Scrophulariaceae in the Holarctic with special reference to the floristic relationships between Eastern Asia and Eastern North America. Ann. Mo. Bot. Gard. 70, 701–712. doi: 10.2307/2398985
Hong, D. Y., Yang, H. B., Jin, C. L., and Noel, H. H. (1998). “Scrophularia,” in Flora of China 18, ed. Z.-Y. Wu (St. Louis, MO: Missouri Botanical Garden Press), 11–20.
Hoorn, C., Straathof, J., Abels, H. A., Xu, Y., Utescher, T., and Dupont-Nivet, G. (2012). A late Eocene palynological record of climate change and Tibetan Plateau uplift (Xining Basin, China). Palaeogeogr. Palaeoclimatol. Palaeoecol. 344-345, 16–38. doi: 10.1016/j.palaeo.2012.05.011
Hou, Q. Z., Meng, L. H., and Yang, H. L. (2008). Pollination ecology of Gentiana siphonantha (Gentianaceae) and a further comparison with its sympatric species. J. Syst. Evol. 46, 554–562. doi: 10.3724/SP.J.1002.2008.07063
Jia, D. R., Abbott, R. J., Liu, T. L., Mao, K. S., Bartish, I. V., and Liu, J. Q. (2012). Out of the Qinghai-Tibet Plateau: Evidence for the origin and dispersal of Eurasian temperate plants from a phylogeographic study of Hippophae rhamnoides (Elaeagnaceae). New Phytol. 194, 1123–1133. doi: 10.1111/j.1469-8137.2012.04115.x
Kalinowski, S. T. (2005). HP-RARE 1.0: A computer program for performing rarefaction on measures of allelic richness. Mol. Ecol. Not. 5, 187–189. doi: 10.1111/j.1471-8286.2004.00845.x
Li, Z. H., Chen, J., Zhao, G. F., Guo, Y. P., Kou, Y. X., Ma, Y. Z., et al. (2012). Response of a desert shrub to past geological and climatic change: A phylogeographic study of Reaumuria soongarica (Tamaricaceae) in western China. J. Syst. Evol. 50, 351–361. doi: 10.1111/j.1759-6831.2012.00201.x
Li, Z. H., Zhang, Q. A., Liu, J. Q., Kallman, T., and Lascoux, M. (2011). The Pleistocene demography of an alpine juniper of the Qinghai-Tibetan Plateau: Tabula rasa, cryptic refugia or something else? J. Biogeogr. 38, 31–43. doi: 10.1111/j.1365-2699.2010.02400.x
Liao, C. Y., Downie, S. R., Yu, Y., and He, X. J. (2012). Historical biogeography of the Angelica group (Apiaceae tribe Selineae) inferred from analyses of nrDNA and cpDNA sequences. J. Syst. Evol. 50, 206–217. doi: 10.1111/j.1759-6831.2012.00182.x
Librado, P., and Rozas, J. (2009). DasSP v5: A software for comprehensive analysis of DNA polymorphism data. Bioinformatics 25:1451. doi: 10.1093/bioinformatics/btp187
Lin, H. Y., Hao, Y. J., Li, J. H., Fu, C. X., Soltis, P. S., Soltis, D. E., et al. (2019). Phylogenomic conflict resulting from ancient introgression following species diversification in Stewartia s.l. (theaceae). Mol. Phylogenet. Evol. 135, 1–11. doi: 10.1016/j.ympev.2019.02.018
Liu, J. Q., Gao, T. G., Chen, Z. D., and Lu, A. M. (2002). Molecular phylogeny and biogeography of the Qinghai-Tibet Plateau endemic Nannoglottis (Asteraceae). Mol. Phylogenet. Evol. 23, 307–325. doi: 10.1016/S1055-7903(02)00039-8
Liu, J. Q., Sun, Y. S., Ge, X. J., Gao, L. M., and Qiu, Y. X. (2012). Phylogeographic studies of plants in China: Advances in the past and directions in the future. J. Syst. Evol. 50, 267–275. doi: 10.1111/j.1759-6831.2012.00214.x
Liu, J. Q., Wang, Y. J., Wang, A. L., Ohba, H., and Abbott, R. J. (2006). Radiation and diversification within the Ligularia–Cremanthodium–Parasenecio complex (Asteraceae) triggered by uplift of the Qinghai-Tibetan Plateau. Mol. Phylogenet. Evol. 38, 31–49. doi: 10.1016/j.ympev.2005.09.010
Ma, S. M., Zhang, M. L., and Sanderson, S. C. (2012). Phylogeography of the rare Gymnocarpos przewalskii (Caryophyllaceae): Indications of multiple glacial refugia in north-western China. Aust. J. Bot. 60, 20–31. doi: 10.1071/BT11055
Mabberley, D. J. (1997). The plant-book: A portable dictionary of the vascular plants. Cambridge: Cambridge University Press.
Manish, K., and Pandit, M. K. (2018). Geophysical upheavals and evolutionary diversification of plant species in the Himalaya. PeerJ 6:e5919. doi: 10.7717/peerj.5919
Mao, K. S., Wang, Y., and Liu, J. Q. (2021). Evolutionary origin of species diversity on the Qinghai-Tibet Plateau. J. Syst. Evol. 59, 1142–1158. doi: 10.1111/jse.12809
Mayrose, I., Zhan, S. H., Rothfels, C. J., Magnuson-Ford, K., Barker, M. S., Rieseberg, L. H., et al. (2011). Recently formed polyploid plants diversify at lower rates. Science 333:1257. doi: 10.1126/science.1207205
Meng, H. H., Gao, X. Y., Huang, J. F., and Zhang, M. L. (2015). Plant phylogeography in arid Northwest China: Retrospectives and perspectives. J. Syst. Evol. 53, 33–46. doi: 10.1111/jse.12088
Miao, Y. F., Herrmann, M., Wu, F. L., Yan, X. L., and Yang, S. L. (2012). What controlled Mid-Late Miocene long-term aridification in Central Asia?–global cooling or Tibetan Plateau uplift: A review. Earth Sci. Rev. 112, 155–172. doi: 10.1016/j.earscirev.2012.02.003
Miao, Y. F., Meng, Q. Q., Fang, X. M., Yan, X. L., Wu, F. L., and Song, C. H. (2011). Origin and development of Artemisia (Asteraceae) in Asia and its implications for the uplift history of the Tibetan Plateau: A review. Quatern. Int. 236, 3–12. doi: 10.1016/j.quaint.2010.08.014
Migliore, J., Baumel, A., Juin, A., and Medail, F. (2012). From Mediterranean shores to central Saharan mountains: Key phylogeographical insights from the genus Myrtus. J. Biogeogr. 39, 942–956. doi: 10.1111/j.1365-2699.2011.02646.x
Mousadik, A., and Petit, R. J. (1996). High level of genetic differentiation for allelic richness among populations of the argan tree Argania spinosa (L.) Skeels endemic to Morocco. TAG. Theor. Appl. Genet. 92, 832–839. doi: 10.1007/BF00221895
Myers, N., Mittermeier, R. A., Mittermeier, C. G., Da Fonseca, G. A. B., and Kent, J. (2000). Biodiversity hotspots for conservation priorities. Nature 403, 853–858. doi: 10.1038/35002501
Nei, M. (1978). Estimation of average heterozygosity and genetic distance from a small number of individuals. Genetics 89, 583–590. doi: 10.1093/GENETICS/89.3.583
Niu, Y., Yang, Y., Zhang, Z. Q., Li, Z. M., and Sun, H. (2011). Floral closure induced by pollination in gynodiocecious Cyananthus delavayi (Campanulaceae): Effects of pollen load and type, floral morph and fitness consequences. Ann. Bot. 108, 1257–1268. doi: 10.1093/aob/mcr224
Phillips, S. J., Anderson, R. P., and Schapire, R. E. (2006). Maximum entropy modeling of species geographic distributions. Ecol. Model. 190, 231–259. doi: 10.1016/j.ecolmodel.2005.03.026
Pons, O., and Petit, R. J. (1996). Measuring and testing genetic differentiation with ordered versus unordered alleles. Genetics 144, 1237–1245. doi: 10.1093/genetics/144.3.1237
Posada, D. (2008). jModelTest: Phylogenetic model averaging. Mol. Biol. Evol. 25, 1253–1256. doi: 10.1093/molbev/msn083
Pritchard, J. K., Stephens, M., and Donnelly, P. (2000). Inference of population structure using multilocus genotype data. Genetics 155, 945–959. doi: 10.1093/genetics/155.2.945
Pritchard, J. K., Wen, X., and Falush, D. (2009). Documentation for structure software: Version 2.3. [WWW document]. Available online at: https://web.stanford.edu/group/pritchardlab/structure.html (accessed August 1, 2009).
Qiu, Y. X., Fu, C. X., and Comes, H. P. (2011). Plant molecular phylogeography in China and adjacent regions: Tracing the genetic imprints of Quaternary climate and environmental change in the world’s most diverse temperate flora. Mol. Phylogenet. Evol. 59, 225–244. doi: 10.1016/j.ympev.2011.01.012
Rogers, A. R. (1995). Genetic evidence for a Pleistocene population explosion. Evolution 49, 608–615. doi: 10.1111/j.1558-5646.1995.tb02297.x
Rogers, A., and Harpending, H. (1992). Population growth makes waves in the distribution of pairwise genetic differences. Mol. Biol. Evol. 9, 552–569. doi: 10.1093/oxfordjournals.molbev.a040727
Scheunert, A., and Heubl, G. (2011). Phylogenetic relationships among new world Scrophularia L. (Scrophulariaceae): New insights inferred from DNA sequence data. Plant Syst. Evol. 291, 69–89. doi: 10.1007/s00606-010-0369-z
Scheunert, A., and Heubl, G. (2014). Diversification of Scrophularia (Scrophulariaceae) in the Western Mediterranean and Macaronesia–Phylogenetic relationships, reticulate evolution and biogeographic patterns. Mol. Phylogenet. Evol. 70, 296–313. doi: 10.1016/j.ympev.2013.09.023
Scheunert, A., and Heubl, G. (2017). Against all odds: Reconstructing the evolutionary history of Scrophularia (Scrophulariaceae) despite high levels of incongruence and reticulate evolution. Org. Divers. Evol. 17, 323–349. doi: 10.1007/s13127-016-0316-0
Schneider, S., and Excoffier, L. (1999). Estimation of past demographic parameters from the distribution of pairwise differences when the mutation rates vary among sites: Application to human mitochondrial DNA. Genetics 152, 1079–1089. doi: 10.1093/GENETICS/152.3.1079
Schoener, T. W. (1968). The Anolis lizards of Bimini: Resource partitioning in a complex fauna. Ecology 49, 704–726. doi: 10.2307/1935534
Shackleton, N. J., Hall, M. A., and Pate, D. (1995). Pliocene stable isotope stratigraphy of site 846. Proc. Ocean Drill. Programs Sci. Results 138, 337–355. doi: 10.2973/odp.proc.sr.138.117.1995
Shi, Y. F., Cui, Z. J., and Su, Z. (2005). The quaternary glaciations and environmental variations in China. Hebei: Hebei Science and Technology Publishing House.
Slatkin, M., and Maddison, W. P. (1989). A cladistic measure of gene flow inferred from the phylogenies of alleles. Genetics 123, 603–613. doi: 10.1093/GENETICS/123.3.603
Spicer, R. A. (2017). Tibet, the Himalaya, Asian monsoons and biodiversity–in what ways are they related? Plant Divers. 39, 5–16. doi: 10.1016/j.pld.2017.09.001
Stamatakis, A., Hoover, P., and Rougemont, J. (2008). A rapid bootstrap algorithm for the RAxML web servers. Syst. Biol. 57, 758–771. doi: 10.1080/10635150802429642
Stebbins, G. L. (1940). The significance of polyploidy in plant evolution. Am. Nat. 74, 54–66. doi: 10.1086/280872
Stiefelhagen, H. (1910). Systematische und Pflanzengeographische Studien zur Kenntnis der Gattung Scrophularia [J]. Bot. Jahrb. Syst. Pflanzengesch. Pflanzengeogr. 44, 406–496.
Sun, H. (2002). Tethys retreat and Himalayas-Hengduanshan Mountains uplift and their significance on the origin and development of the Sino-Himalayan elements and alpine flora. Acta Bot. Yunnanica 24, 273–288.
Sun, J. M., Ding, Z. L., and Liu, T. S. (1998). Desert distributions during the glacial maximum and climatic optimum: Example of China. Episodes 21, 28–31. doi: 10.18814/epiiugs/1998/v21i1/005
Sun, J. M., Zhang, L. Y., Deng, C. L., and Zhu, R. X. (2008). Evidence for enhanced aridity in the Tarim Basin of China since 5.3 Ma. Quat. Sci. Rev. 27, 1012–1023. doi: 10.1016/j.quascirev.2008.01.011
Sun, Y. S., Wang, A. L., Wan, D. S., Wang, Q., and Liu, J. Q. (2012). Rapid radiation of Rheum (Polygonaceae) and parallel evolution of morphological traits. Mol. Phyl. Evol. 63, 150–158. doi: 10.1016/j.ympev.2012.01.002
Swets, J. A. (1988). Measuring the accuracy of diagnostic systems. Science 240, 1285–1293. doi: 10.1126/science.3287615
Tajima, F. (1989). Statistical method for testing the neutral mutation hypothesis by DNA polymorphism. Genetics 123, 585–595. doi: 10.1093/GENETICS/123.3.585
Tang, L. Z., Wang, L. Y., Cai, Z. Y., Zhang, T. Z., Ci, H. X., Lin, G. H., et al. (2010). Allopatric divergence and phylogeographic structure of the plateau zokor (Eospalax baileyi), a fossorial rodent endemic to the Qinghai-Tibetan Plateau. J. Biogeogr. 37, 657–668. doi: 10.1111/j.1365-2699.2009.02232.x
Udupa, S., and Baum, M. (2001). High mutation rate and mutational bias at (TAA)n microsatellite loci in chickpea (Cicer arietinum L.). Mol. Genet. Genom. 265, 1097–1103. doi: 10.1007/s004380100568
Wang, A. L., Schluetz, F., and Liu, J. Q. (2008). Molecular evidence for double maternal origins of the diploid hybrid Hippophae goniocarpa (Elaeagnaceae). Bot. J. Linn. Soc. 156, 111–118. doi: 10.1111/j.1095-8339.2007.00729.x
Wang, A. L., Yang, M. H., and Liu, J. Q. (2005). Molecular phylogeny, recent radiation and evolution of gross morphology of the rhubarb genus Rheum (Polygonaceae) inferred from chloroplast DNA trnL-F sequences. Ann. Bot. 96, 489–498. doi: 10.1093/aob/mci201
Wang, H., Qiong, L., Sun, K., Lu, F., Wang, Y. G., Song, Z. P., et al. (2010). Phylogeographic structure of Hippophae tibetana (Elaeagnaceae) highlights the highest microrefugia and the rapid uplift of the Qinghai-Tibetan Plateau. Mol. Ecol. 19, 2964–2979. doi: 10.1111/J.1365-294X.2010.04729.X
Wang, Q., Abbott, R. J., Yu, Q. S., Lin, K., and Liu, J. Q. (2013). Pleistocene climate change and the origin of two desert plant species, Pugionium cornutum and Pugionium dolabratum (Brassicaceae), in northwest China. New Phytol. 199, 277–287. doi: 10.1111/nph.12241
Wang, R. H. (2015). Phylogeny and biogeography of Scrophularia and phylogeography of S. Incisa complex [D]. Hangzhou: Zhejiang University.
Wang, R. H., Chen, C., Ma, Q., Li, P., and Fu, C. X. (2014). Development of microsatellite loci in Scrophularia incisa (Scrophulariaceae) and cross-amplification in congeneric species. Appl. Plant Sci. 2:1300077. doi: 10.3732/apps.1300077
Wang, R. H., Xia, M. Q., Tan, J. B., Chen, C., Jin, X. J., Li, P., et al. (2018). A new species of Scrophularia (Scrophulariaceae) from Hubei. China. Phytotaxa. 350, 001–014. doi: 10.11646/phytotaxa.350.1.1
Wang, Y., Liu, Y. F., Liu, S. B., and Huang, H. W. (2009). Molecular phylogeny of Myricaria (Tamaricaceae): Implications for taxonomy and conservation in China. Bot. Stu. 50, 343–352. doi: 10.1016/j.aquabot.2009.01.002
Warren, D. L., Glor, R. E., and Turelli, M. (2010). ENMTools: A toolbox for comparative studies of environmental niche models. Ecography 33, 607–611. doi: 10.1111/j.1600-0587.2009.06142.x
Wen, J., Zhang, J. Q., Nie, Z. L., Zhong, Y., and Sun, H. (2014). Evolutionary diversifications of plants on the Qinghai-Tibetan Plateau. Front. Genet. 5:4. doi: 10.3389/fgene.2014.00004
Wen, Z. B., Li, Y., Zhang, H. X., Meng, H. H., Feng, Y., and Shi, W. (2016). Species-level phylogeographical history of the endemic species Calligonum roborovskii and its close relatives in Calligonum section Medusa (Polygonaceae) in arid north-western China. Bot. J. Linn. Soc. 180, 542–553. doi: 10.1111/boj.12381
Willis, J. C. (1973). A dictionary of the flowering plants and ferns, 8th Edn. Cambridge: University Press.
Wu, L. L., Cui, X. K., Milne, R. I., Sun, Y. S., and Liu, J. Q. (2010). Multiple autopolyploidizations and range expansion of Allium przewalskianum Regel. (Alliaceae) in the Qinghai-Tibetan Plateau. Mol. Ecol. 19, 1691–1704. doi: 10.1111/j.1365-294X.2010.04613.x
Wu, Z. Y., and Wu, S. G. (1996). “A proposal for a new floristic kingdom (realm)the E. Asiatic kingdom, its delimitation and characteristics,” in Proceedings of the 1st International Symposium on Floristic Characteristics and Diversity of East Asian Plants, eds A. L. Zhang and S. G. Wu (Beijing: China Higher Education Press), 3–42.
Xie, L., Wen, J., and Li, L. Q. (2011). Phylogenetic analyses of Clematis (Ranunculaceae) based on sequences of nuclear ribosomal ITS and three plastid regions. Syst. Bot. 36, 907–921. doi: 10.1600/036364411X604921
Xu, L., Cao, M. T., Wang, Q. C., Xu, J. H., Liu, C. L., Ullah, N., et al. (2022). Insights into the plateau adaptation of Salvia castanea by comparative genomic and WGCNA analyses. J. Adv. Res. doi: 10.1016/j.jare.2022.02.004
Xu, T. T., Abbott, R. J., Milne, R. I., Mao, K. S., Du, F. K., Wu, G. L., et al. (2010). Phylogeography and allopatric divergence of cypress species (Cupressus L.) in the Qinghai–Tibetan Plateau and adjacent regions. BMC Evol. Biol. 10:194. doi: 10.1186/1471-2148-10-194
Xu, W. Q., Losh, J., Chen, C., Li, P., Wang, R. H., Zhao, Y. P., et al. (2018). Comparative genomics of figworts (Scrophularia, Scrophulariaceae), with implications for the evolution of Scrophularia and Lamiales. J. Syst. Evol. 57, 55–65. doi: 10.1111/jse.12421
Yang, D. F., Du, X. H., Yang, Z. Q., Liang, Z. S., Guo, Z. X., and Liu, Y. (2014). Transcriptomics, proteomics, and metabolomics to reveal mechanisms underlying plant secondary metabolism. Eng. Life Sci. 14, 456–466. doi: 10.1002/elsc.201300075
Yang, F. S., Wang, X. Q., and Hong, D. Y. (2003). Unexpected high divergence in nrDNA ITS and extensive parallelism in floral morphology of Pedicularis (Orobanchaceae). Plant Syst. Evol. 240, 91–105. doi: 10.1007/s00606-003-0005-2
Yang, S. J., Dong, H. L., and Lei, F. M. (2009). Phylogeography of regional fauna on the Tibetan Plateau: A review. Prog. Nat. Sci. 19, 789–799. doi: 10.1016/j.pnsc.2008.10.006
Yu, Y., Blair, C., and He, X. J. (2020). RASP 4: Ancestral State reconstruction tool for multiple genes and characters. Mol. Biol. Evol. 37, 604–606. doi: 10.1093/molbev/msz257
Yue, J. P., Sun, H., David, B. A., Li, J. H., Al-Shehbaz, I. A., and Ree, R. (2009). Molecular phylogeny of Solms-laubachia (Brassicaceae) s.l., based on multiple nuclear and plastid DNA sequences, and its biogeographic implications. J. Syst. Evol. 47, 402–415. doi: 10.1111/j.1759-6831.2009.00041.x
Zachos, J. C., Shackleton, N. J., Revenaugh, J. S., Pälike, H., and Flower, B. P. (2001). “The climatic consequences of a rare orbital anomaly at the Oligocene/Miocene boundary (23Ma),” in Proceedings of the Earth System Processes 2001: GSA/GSL Global Meeting (Edinburgh: Geological Society of America), 94.
Zhang, L. Q., Zhu, T. T., Qian, F., Xu, J. W., Dorje, G., Zhao, Z. L., et al. (2014). Iridoid glycosides isolated from Scrophularia dentata Royle ex Benth. and their anti-inflammatory activity. Fitoterapia 98, 84–90. doi: 10.1016/j.fitote.2014.07.005
Zhang, J. Q., Meng, S. Y., Wen, J., and Rao, G. Y. (2014). Phylogenetic relationships and character evolution of Rhodiola (Crassulaceae) based on nuclear ribosomal ITS and plastid trnL–F and psbA–trnH sequences. Syst. Bot. 39, 441–451. doi: 10.1600/036364414X680753
Zhang, L. Q., Yang, Z., Jia, Q., Dorje, G., Zhao, Z., Guo, F. J., et al. (2013). Two new phenylpropanoid glycosides with interesterification from Scrophularia dentata Royle ex Benth. J. Mol. Struct. 1049, 299–302. doi: 10.1016/j.molstruc.2013.05.039
Zhang, M. L., and Fritsch, P. W. (2010). Evolutionary response of Caragan (Fabaceae) to Qinghai-Tibetan Plateau uplift and Asian interior aridification. Plant Syst. Evol. 288, 191–199. doi: 10.1007/s00606-010-0324-z
Zhang, Z. S., Flatøy, F., Wang, H. J., Bethke, I., Bentsen, M., and Guo, Z. T. (2012). Early eocene asian climate dominated by desert and steppe with limited monsoons. J Asian Earth Sci. 44, 24–35. doi: 10.1016/j.jseaes.2011.05.013
Zhou, X., Zhang, Z. C., Huang, Y. B., Xiao, H. W., Wu, J. J., Qi, Z. C., et al. (2021). Conservation genomics of wild red sage (Salvia miltiorrhiza) and its endangered relatives in China: Population structure and interspecific relationships revealed from 2b-RAD data. Front. Genet. 12:688323. doi: 10.3389/FGENE.2021.688323
Keywords: allopatric speciation, aridification, divergence, phylogeography, Scrophularia incisa complex
Citation: Wang R-H, Yang Z-P, Zhang Z-C, Comes HP, Qi Z-C, Li P and Fu C-X (2022) Plio-Pleistocene climatic change drives allopatric speciation and population divergence within the Scrophularia incisa complex (Scrophulariaceae) of desert and steppe subshrubs in Northwest China. Front. Plant Sci. 13:985372. doi: 10.3389/fpls.2022.985372
Received: 03 July 2022; Accepted: 19 August 2022;
Published: 21 September 2022.
Edited by:
Tingshuang Yi, Kunming Institute of Botany (CAS), ChinaReviewed by:
Hong-Xiang Zhang, Xinjiang Institute of Ecology and Geography (CAS), ChinaKangshan Mao, Sichuan University, China
Copyright © 2022 Wang, Yang, Zhang, Comes, Qi, Li and Fu. This is an open-access article distributed under the terms of the Creative Commons Attribution License (CC BY). The use, distribution or reproduction in other forums is permitted, provided the original author(s) and the copyright owner(s) are credited and that the original publication in this journal is cited, in accordance with accepted academic practice. No use, distribution or reproduction is permitted which does not comply with these terms.
*Correspondence: Zhe-Chen Qi, zqi@zstu.edu.cn; Pan Li, panli_zju@126.com